- Review
- Open access
- Published:
Targeting lipid droplets and lipid droplet-associated proteins: a new perspective on natural compounds against metabolic diseases
Chinese Medicine volume 19, Article number: 120 (2024)
Abstract
Background
Lipid droplet (LD) is a metabolically active organelle, which changes dynamically with the metabolic state and energy requirements of cells. Proteins that either insert into the LD phospholipid monolayer or are present in the cytoplasm, playing a crucial role in lipid homeostasis and signaling regulation, are known as LD-associated proteins.
Methods
The keywords “lipid droplets” and “metabolic diseases” were used to obtain literature on LD metabolism and pathological mechanism. After searching databases including Scopus, OVID, Web of Science, and PubMed from 2013 to 2024 using terms like “lipid droplets”, “lipid droplet-associated proteins”, “fatty liver disease”, “diabetes”, “diabetic kidney disease”, “obesity”, “atherosclerosis”, “hyperlipidemia”, “natural drug monomers” and “natural compounds”, the most common natural compounds were identified in about 954 articles. Eventually, a total of 91 studies of 10 natural compounds reporting in vitro or in vivo studies were refined and summarized.
Results
The most frequently used natural compounds include Berberine, Mangostin, Capsaicin, Caffeine, Genistein, Epigallocatechin-3-gallate, Chlorogenic acid, Betaine, Ginsenoside, Resveratrol. These natural compounds interact with LD-associated proteins and help ameliorate abnormal LDs in various metabolic diseases.
Conclusion
Natural compounds involved in the regulation of LDs and LD-associated proteins hold promise for treating metabolic diseases. Further research into these interactions may lead to new therapeutic applications.
Graphical Abstract
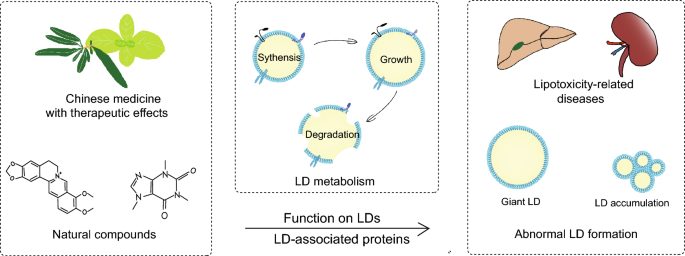
Introduction
The prevalence of metabolic diseases, including obesity, dyslipidemia, type 2 diabetes mellitus (T2DM), and non-alcoholic fatty liver disease (NAFLD), is escalating yearly, posing a serious threat to human health [1]. According to the latest epidemiology, overweight rates in adults reached 42.4% and 59% in the United States and Europe, respectively [2]. The global incidence of NAFLD has reached 32.4% by 2021 [3]. The optimal treatment for these diseases involves improving lifestyle and dietary habits, yet the outcome is poor due to a lack of patient adherence [4].
As an active organelle, lipid droplet (LD) varies dynamically with the metabolic state and energy requirements of cells. Early research established that LDs are crucial for lipid metabolism, with abnormalities often leading to lipotoxicity-related diseases, like diabetes, and fatty liver diseases [5]. LD-associated proteins, also known as LD proteins, are decorative proteins of phospholipid monolayers, including lateral proteins and gross membrane proteins which employ a single topology [6]. Primary studies of LD-associated proteins referred to classical protein families like perilipins (PLINs), which were found to prevent lipolysis of LDs [7,8,9,10]. Subsequently, more molecules are gradually being recognized as LD-associated proteins, like Cell death-induced DNA fragmentation factor 45-like effector (CIDE), hypoxia inducible gene 2 (HIG2), etc. [11, 12]. LD-associated proteins are involved in various physiological activities such as LD biogenesis, growth, fusion, and lipolysis, thus affecting lipid homeostasis [13]. Therefore, targeting the regulation of LDs and LD-associated proteins may offer novel approaches for the therapy [14].
Massive basic or clinical studies have confirmed that traditional Chinese medicine could remarkably confront metabolic disorders by improving lipid metabolism. However, traditional Chinese medicine have complex components and their pharmacological mechanism have not been thoroughly elucidated, resulting in limited application. Moreover, the potential role in regulating LDs remains unknown. The keywords “lipid droplets” and “metabolic diseases” were used to obtain literature on LD metabolism and pathological mechanisms. After searching databases including Scopus, OVID, Web of Science, and PubMed from 2013 to 2024 using terms like “lipid droplets”, “lipid droplet-associated proteins”, “fatty liver disease”, “diabetes”, “diabetic kidney disease”, “obesity”, “atherosclerosis”, “hyperlipidemia”, “natural drug monomers” and “natural compounds”, we identified the most common natural compounds in about 954 articles. Eventually, a total of 91 studies of 10 natural compounds reporting in vitro or in vivo studies were refined and summarized to elucidate the mechanism by which natural compounds affect LDs and LD-associated proteins. We aim to provide a reference for further research on the regulatory mechanism of natural compounds and their effective management of metabolic diseases.
Connection between LD dynamics and LD-associated proteins
LD primarily consists of a core formed by triacylglycerol (TAG) and cholesterol ester (CE), which are enveloped by an amphiphilic phospholipid monolayer containing numerous embedded proteins on the surface [15]. In addition to storing and regulating lipid utilization [16], LD serves as a reservoir for proteins [17]. The proteomics of LD has revealed over 200 protein components, some of them fixate only in LDs, while others are also present in other subcellular compartments [18]. As a metabolically active organelle, the size, morphology, number, lipid composition and protein composition of LD can change according to the metabolic situation of the organism. The dynamics of LD include LD biogenesis, expansion, fusion, degradation, and contacts with other cellular organelles [19]. The orderly unfolding of these processes depends on LD-associated proteins.
LD-associated proteins
Various LD-associated proteins can be generally classified according to their functions as follows: enzymes that catalyze lipid metabolism, histones that bind nucleic acids to LDs, ribosomal proteins that exist in protein synthesis in prokaryotes, proteins from protein degradation, proteins with signaling capabilities, membrane transport proteins that interact with other organelles, dynamics related proteins such as microtubules and skeletal proteins responsible for movement, LD resident proteins that are directly targeted to LDs with subcellular structural characteristics, etc. [20].
Protein targeting occurs in multiple biological processes in LD including LD biogenesis, growth, degradation, etc. Based on their targeting pathways, LD-associated proteins can be subdivided into two classes. The first class, “ERTELLED” (classical class I), consists of proteins inserted into the endoplasmic reticulum (ER) membrane that relocate to the LD surface through ER-LD contact. The second class, “CYTELLED” (classical class II), comprises proteins translated in the cytoplasm that directly target the LD surface [21, 22].
ERTELLED proteins generally comprise hydrophobic membrane-embedded sequences that acquire a hairpin configuration in the ER bilayer. This class includes esteryol coenzyme A synthase (e.g. Acyl-CoA synthetase long-chain family member 3 (ACSL3), ACSL5) [23], triglyceride lipase (e.g. adipose triglyceride lipase/Patatin-like phospholipase domain-containing protein 2 (ATGL/PNPLA2) [24], acyltransferases (e.g. GPAT4, LPCAT) [25], LD-associated hydrolase [26], LD assembly factor 1 (LDAF1, also known as TMEM159) [27], and UBX structural domain-containing protein 8 (UBXD8) [28], etc.
CYTELLED proteins are soluble proteins with several LD-binding amphipathic helix motifs, including the PLIN family, comparative gene identification-58 (CGI-58, also known as ABHD5) and Phosphocholine cytidylyltransferase (PCYT or CCT) [29,30,31]. Among these, the PLINs are the main LD-associated proteins [32]. PLIN1 and PLIN4 are mainly expressed in White adipose tissue (WAT). PLIN5 is generally expressed in the liver, heart, BAT and skeletal muscle. Meanwhile, PLIN2 and PLIN3 are widely expressed in various tissues [33].
LD Biogenesis
Present studies supposed that the synthesis of neutral lipids plays a major part in LD biogenesis. The neutral lipids synthesis from activated fatty acids (FAs) represents the first step of LD biogenesis. If FA esters to diacylglycerol (DAG), it is further catalyzed by diacylglycerol acyltransferases to produce TAG. Similarly, if FA esterifies to sterols (e.g. cholesterol), they are catalyzed by cholesterol o -acyltransferases to CE afterward [34].
ER-LD contact is known to make sense for LD formation [35]. Seipin, an ER protein that mediates ER-LD contacts, determines the site where LDs form in the ER and interacts with LDAF1 to form the LDAF1-Seipin complex [36]. The complex co-purifies with TAG, followed by TAG elution by membrane phospholipids, forming LDs [37]. After LD formation, LDAF1 splits from Seipin, turning to the LD surface. While lacking the complex, only high levels of TAG (4% or above) can facilitate LD formation [38]. Seipin also works as a scaffolding protein to recruit enzymes like 1-acylglycerol-3-phosphate O-acyltransferase 2 (AGPAT2) and lipoprotein 1 (Lipin-1), and restrains palmitic acid (PA) levels by reducing Glycerol-3-phosphate acyltransferase (GPAT) [39, 40] (Fig. 1).
LD biogenesis. Activated fatty acids produce neutral lipids in TAG synthesis. Wrapped by the phospholipid molecule shell deriving from the ER leaflets, the neutral lipids sprout into oil lenses and grow larger. When the superficial tension attains a certain level, the budding LD flips form a mature LD
Nucleus LDs (nLDs) are commonly found in cultured cells of liver and hepatic origin [41, 42], with a higher ratio of CE and TAG compared to cytoplasmic LDs (cLDs). Interestingly, although Seipin is an essential protein for cLD formation, the knockdown of Seipin may increase the amount of nLDs in the experimental strains instead [43].
LD growth
After initial biogenesis, LD tends to expand by fusion between LDs and local lipid synthesis (Fig. 2A). LD fusion occurs through LD-LD coalescence or LD-LD lipid transfer [44]. LD coalescence occurs when the stability of phospholipid monomolecular membrane of LDs decreases due to a reduction in phosphatidylcholine (PC) or the aggregation of fusogenic PA [31]. Phosphocholine cytidylyltransferase (PCYT or CCT) is a key rate-limiting enzyme in the Kennedy pathway of PC synthesis. Among them, PCYT1A (CCT1) can be transported directly from the cytoplasm to the surface of LDs [45]. The knockdown of PCYT1A results in increased production of LDs, while its activation promotes the maintenance of LD homeostasis [31, 46].
LD growth and LD degradation. A 1. When LDs contact with ER, adipogenic enzymes catalyze the TAG synthesis. 2&3. Both LD fusion and lipid transfer between LDs render the growth. B 1. Macroautophage is the process of formation of autophagosome by double-membrane vacuoles before fusion with lysosomes. 2. Chaperon-mediated autophagy (CMA) refers to lysosomal selective capture of LDs containing the KFERQ motif. 3. Direct lysosomal degradation of LDs is microautophagy
CIDE can moderate the formulation of oversized LDs in adipocytes by mediating LD-LD lipid transfer. CIDEs locate to LDs through a carboxy-terminal amphiphilic helix to generate a dimer. The dimer diffuses on the LD surface and engages with another LD containing CIDE. The presence of CIDE on both LD surfaces promotes the formation of stable trans-organelle oligomers, which provide channels for lipid transfer [47]. This process allows neutral lipids to migrate from smaller LDs to larger LDs, while protein transfer does not occur [48]. In human hepatocytes, CIDEB promotes TAG transfer, but this catalytic role relies on the expression of CIDEC or CIDEA [49].
In addition, neutral lipid synthase-mediated local lipid synthesis is involved in the growth of LDs. The previously mentioned membrane bridge in LD-ER contact enables adipogenic enzymes like diacylglycerol acyltransferase 2 (DGAT2) and GPAT4 from ER to the LD surface, catalyzing the local synthesis of neutral lipids [50]. Lipin-1 (also known as PAH1) with phosphatidic acid phosphatase (PAP) activity, co-localizing with other proteins like PLIN2 and PLIN3, catalyzes PA into DAG [51]. Eventually, LD formation arises at the ER-LD interface [52].
Contacts with other organelles
Recent research has revealed that LDs inevitably come into contact with other organelles when performing biological functions, through the fusion with phospholipid monolayers and variations of LD-associated proteins [53, 54]. LD-ER contact triggers LD generation and LD-associated protein targeting; LD-LD contact causes LD enlargement; LD-nuclear membrane contact lays the basis for nLD formation; LD-lysosome contact provides conditions for autophagy. In addition, LDs also seem to contact with mitochondria and peroxisome.
During nutrient deprivation, LD-mitochondrial contact function as sites of lipogenesis or catabolism [55, 56]. Although the specifies of LD-mitochondrial haven’t been clarified yet, studies have found that PLIN5 overexpression in BAT to induces mitochondrial recruitment at the LD periphery [57], and PLIN1 interacts with mitochondrial protein 2 [58]. A recent review summarized LD-associated protein interactions that mediate LD-mitochondrial contact: PLIN5-fatty acid transporter protein (FATP4) and Mitofusin2 (MFN2)-Heat shock cognate 71 kDa protein (HSC70)-PLIN1 for fatty acid oxidation; ADP-ribosylation factor related protein 1 (ARFRP1)-Synaptosome-associated protein 23 (SNAP23) and mitoguardin 2 (MIGA2)-Vacuolar Protein Sorting 13D (VPS13D) for LD growth [59].
Similar to the function of mitochondria, the peroxisome is the only setting of β -oxidation in yeast, along with branched-chain fatty acids and β -oxidation of very long-chain fatty acids in humans [60]. The molecular basis for the fusion between the outer layer of peroxisomal phospholipid leaflets and the LD membrane still requires clarification [61].
LD degradation
LD degradation frequently occurs, though not exclusively, following the completion of biological processes. The most common form of degradation for LDs is lipolysis, which requires ATGL /PNPLA2 and its activator CGI-58, hormone-sensitive triglyceride lipase (HSL), and monoacylglycerol lipase (MAGL) [62,63,64], etc. Moreover, ATGL is activated via the patatin structural domain interacting with CGI-58, as well as inhibited by CIDEC. During nutrient deprivation, LDs can also degrade by lipophagy, a selective autophagic procedure that transports part or the entire LDs to lysosomes, undergoing bulk degradation by hydrolytic enzymes [65].
The PLIN family, governed by sterol regulatory element binding protein (SREBP), liver X receptor (LXR) and peroxisome proliferators-activated receptor (PPAR), affects LD degradation by regulating the efficiency of lipase entry to the surface of LDs [66]. For instance, chaperone-mediated autophagy (CMA) degradation of PLIN2/3 promotes the entry of ATGL and autophagy proteins into the surface of LDs. The Rab proteins, consisting of around 70 small GTPases, modulate cytoskeletal motility and are also involved in the autophagic process [67]. Rab7 regulates the interaction between lysosomes and autophagosomes through members of the homotypic fusion and protein sorting (HOPS) tethering complex and soluble NSF attachment protein receptor (SNARE) proteins [68] (Fig. 2B).
The role of LDs in relevant diseases
LDs play a vital part in lipid metabolism and signaling pathways. Abnormalities of LD-associated proteins leads to dysregulation of LD dynamics, resulting in significant disorders (Fig. 3). The available evidence indicates that LDs and LD-associated proteins are closely related to metabolic diseases.
Abnormal LD formation in metabolism-related diseases. The FASN-mediated lipid synthesis system and CIDE-mediated LD fusion promote the generation of giant LDs. Moreover, the expression of ATGL, CGI8, Rab7/18, ACOX, CPT1, ABCA1, and ABCG1 is downregulated, inhibiting lipolysis and β-oxidation, resulting in LD deposition
Metabolic diseases
NAFLD, is a spectrum of chronic liver diseases that includes simple hepatic steatosis, nonalcoholic steatohepatitis, fibrosis and cirrhosis [69]. During steatosis, excessive triglycerides are stored in LDs [70]. The continuous generation of LDs, frequent LD fusion and impaired LD autophage contribute to LD accumulation [71, 72]. Furthermore, extremely large LDs cluster in hepatocytes [73].
In mouse models, CIDEC, CIDEA and PLIN2 are highly upregulated in hepatocytes [74, 75], while the same changes of CIDEC occur in patients [76]. PLIN2 may display an anti-lipolytic impact by disrupting the action of ATGL on LDs [77]. Knockdown of PLIN5 in high-fat-diet (HFD) mice results in reduced steatosis and fibrosis in the liver, suppression of SREBP1 and its downstream fatty acid synthase (FASN),and inactivation of adenosine 5’-monophosphate-activated protein kinase (AMPK) [78]. Besides, PNPLA3 (I148M) accumulation on the LD surface may segregate CGI58, thus promoting lipolysis [79, 80]. As for the Rab family, both Rab18, which has a regulatory role in lipolysis, and Rab7, which is related to autophagy are decreased in steatotic livers [81]. Serum levels of very low-density lipoprotein (VLDL) and TAG from VLDL are reduced in mice with CIDEB loss, while TAG levels in liver are increased [82].
Diabetes is also closely related to the homeostasis of LDs. Dai et al. found that mice transplanted with human islets developed more LDs in islet cells after a HFD [83], while hypertrophic LDs stack in adipocytes [59]. Hyperglycemia induce a significant fraction of lipophagy, ultimately resulting in LD accumulation and inflammation [84]. Similarly, renal biopsies from patients with diabetic nephropathy (DN) showed increased lipid deposition and LDs [85], which may be a compensatory protective pathway against lipotoxicity [86]. Resulting from increased PLIN2, decreased autophagic flux and excessive PPARδ activated by lysophosphatidylcholine, LD accumulation contributes to the decline in rapid renal function in DN [87].
Insulin secretion may be regulated by LD degradation. Lipolysis-generated FA promotes insulin secretion by prompting the PPARδ pathway and adenosine 5′-triphosphate production in mitochondria [88]. Local FA activates cell surface receptors (e.g. FAR1) and enhances insulin secretion [88]. Meanwhile, lipolysis-generated 1-monoacylglycerols (1-MAG) also stimulates insulin granule cytosolic action [89]. Unlike healthy people, patients with T2DM fail to undergo lipolysis induced by glucose for a currently unknown mechanism. However, present studies have confirmed that when the key enzymes for lipolysis (e.g. ATGL) become defective, palmitoylation of insulin synthesis protein 1a (STX1a) decreases, accelerating the degradation of STX1a and lowering insulin secretion [90].
Renal diseases
When acute kidney injury (AKI) occurs, there is a deficiency of AMPKα with LD accumulation in the renal tubules [91]. In the plasma of patients with primary focal segmental glomerulosclerosis, the circulating permeability factor induces LD accumulation and overexpression of PLIN2 (a marker of renal fibrosis) [92, 93]. Fibrosis in the renal interstitium features increases LDs in proximal tubular cells, and the expression of PPARα, carnitine palmitoyltransferases 1 (CPT1), acyl-Coenzyme A oxidase 1 (ACOX1), PPARγ coactivator 1α (PGC-1α), and fatty acid binding protein 4 (FABP4) elevates [94].
AKI is a substantial risk for the occurrence and development of chronic kidney disease (CKD) [95]. In CKD models, the expression levels of CPT1, ACOX1, and L-FABP decrease significantly [96, 97]. Instead, the expression levels of LDL receptor (LDLR) and SREBP-2 increase significantly [96, 97]. CPT1, ACOX1, and L-FABP are the crucial proteins in fatty acid β-oxidation pathway, while LDLR and SREBP-2 are associated with cholesterol uptake. Reduced catabolism and excessive sterol intake result in enhanced neutral lipid content from LDs and eventually lead to the formation of giant LDs [98, 99].
Cardiovascular diseases
In atherosclerosis (AS), lipids, primarily CE, increase the phagocytic load of macrophages, leading to the formation of more foam cells. Compared to normal areas of arteries at the same level, the high expression of PLIN2 in plaques induces the overexpression of pro-inflammatory cytokines [100, 101]. Additionally, PLIN2 aggravates the accumulation of CE in macrophages by restricting cholesterol efflux [102]. CIDEB-knockout mice show reduced plasma LDL and cholesterol levels, but increased liver cholesterol, which may result from the increased expression of LDLR and Acyl coenzyme A-cholesterol acyltransferase (ACAT1) [103]. Although HSL has a broad substrate specificity, it vanishes in human AS progression [104].
Clinical studies have shown that PLIN5 increased during heart attacks, similar to the high-sensitivity cardiac troponin I, thus may serve as a biomarker [105]. In addition, PLIN5, previously mentioned, functions in mediating LD-mitochondria contact, may provide new avenues for the therapy of myocardial ischemia–reperfusion injury (IRI). In the mouse models, inhibition of PLIN5 can promote cardiomyocyte proliferation and activate the PPAR signaling pathway, reduce the expression of PPARγ but raise the expression of PPARα [106]. Above all, increased PLINs may be the reason for more LDs, while various lipotoxic intermediates leading to excessive deposition of LDs in vesicles in the myocardium and eventually causing cardiac steatosis [107].
Cancer
Lipid mobilization of LDs provides additional energy for drastically proliferating cancer cells: the FAs from core catabolism enter the mitochondria for oxidative energy supply [108]. LDs can also ensure cancer cell survival by reducing lipotoxicity via storing excess lipids in the inflammatory microenvironment [109]. ACSL4 can increase the contents of TAG and LDs in hepatocellular carcinoma cells [110]. ACSL3, which is required for exogenous fatty acid lipogenesis, expresses more to induce LD accumulation in renal cell carcinoma [111,112,113]. The synthesis of TAG, along with CIDEC and PLIN3, appears to be highly expressed with the decrease of CIDEB. The lipogenic proteins like ACSL4 and ACSL4 are upregulated, while CIDEB involved in TAG transfer is downregulated, which ultimately results in LD accumulation. What’s more, LDs may remove misfolded proteins by regulating fatty acids, the mechanism of which remains to be investigated [114].
LD-associated proteins have diagnostic, therapeutic and prognostic values in cancer [115], as exemplified by PLINs. PLIN2 has enhanced expression in urological cancers [116], and also has reference in the diagnosis of breast cancer staging and colorectal cancer [117, 118]. PLIN1 has potential to inhibit breast cancer [119]. PLIN4 overexpresses in adriamycin-resistant cells, which can be targeted to eliminate chemoresistance [119].
Other diseases
Congenital lipodystrophy (CGI) is a group of heterogeneous disorders defined by a particular lack of fatty tissue with ectopic steatosis, dyslipidemia, and insulin resistance (IR) [120]. The supplementation of leptin may alleviate related symptoms. CGI is a scarce autosomal recalculant disease, relevant to the gene deletions of AGPAT2 (BSCL1), Seipin [121].
Some common pathogens usually metabolize by means of LDs in host cells. The hepatitis B virus invokes hepatic steatosis in the mouse model by targeting its core protein to LDs and enhancing LD-ER contact. This is achieved through NS5A binding to Rab18, which promotes LD generation and LD-associated protein targeting [122, 123]. Knockdown of PLIN3 attenuates this steatosis [124]. In addition to mediating pathogen infection, LDs also play an immunological role. For instance, the translation of viperin (an innate immune protein) posts on LDs [125].
Links between natural compounds and LDs
Studies on natural compounds associated with lipid regulation have mainly focused on signaling pathways like AMPK, mammalian target of rapamycin (mTOR) and PPAR. Some downstream targets of these signaling pathways, which are involved in lipid metabolism, are also discovered to be localized on LDs. These targets including GPAT4, DGAT1, DGAT2, CPT1, and ATGL, are regarded as LD-associated proteins [53, 54, 126, 127]. Exploring the connection between natural compounds and LDs is of great significance for their clinical use and further development. Based on the current studies, we have summarized the mechanisms of lipid-lowering and regulation of LD homeostasis by natural compounds from the perspective of LD-associated proteins. We aim to provide new references for the clinical utilization and development of theses natural compounds (Table 1).
Berberine (BBR)
BBR (Fig. 4A), is an isoquinoline alkaloid primarily enriched in the rhizomes of Berberis spp., Coptis, and cortex of Phellodendron [128]. BBR is originally used to treat diarrhea and has since been found to have a positive therapeutic effect on glycolipid metabolism disorders. BBR can attenuate hepatic TAG accumulation caused by overnutrition through reducing the expression of stearoyl coenzyme a desaturase 1 (SCD1) [129], fatty acid uptake-related proteins (e.g. FABP1, CD36), and fatty acid oxidation-related proteins (e.g. CPT1a) via the AMPK-SREBP1c pathway [130]. Wang, et al. found that BBR intake decreased liver fat content while elevating muscle lipid accumulation in black snapper fed with an HFD. The promotion of lipid mobilization by BBR may be related to the regulation of proteins associated with stearoyl coenzyme a desaturase (ACCα) HSL, and lipoprotein lipase (LPL) [131]. Based on the studies above, by restricting SCD1/CD36-related fatty acid synthesis and enhancing HSL/LPL-related lipolysis, BBR reduces LD content by restricting the enlargement of neutral fat nuclei.
Chemical structures and mechanism of natural compounds. (A) BBR; (B) MGF; (C) CAP; (D) CAF; (E) GEN; (F) EGCG; (G) CGA; (H) BET; (I) GIN; (J) RES. All the chemical structures were obtained from pubchem: https://pubchem.ncbi.nlm.nih.gov/
Moreover, BBR has been reported to reduce the number and surface area of LDs in porcine oocytes at the IVM stage, stimulating miR-192 to downregulate SREBP1 and PPARγ [132]. Nevertheless, the detailed downstream effects of SREBP1 and PPARγ were not mentioned. Studies in colon cancer cells revealed that BBR inhibited lipogenesis and LD accumulation by promoting the ubiquitinated degradation of promyelocytic leukemia zinc finger-mediated SREBP-cleavage-activating protein [133]. The findings suggest that BBR may regulate LD through the PPARγ-SREBP1 pathway.
Mangostin (MGF)
MGF is a botanical isoflavone (Fig. 4B), mainly existing in Iris unguicularis, Mangifera indica, Cyclopia genitives, Salacia chinensis, Bombax ceiba and Anemarrhena asphodeloides [134]. MGF is utilized to reduce serum TAG, FA, and improve blood lipid profiles [135]. Studies in NAFLD models have shown that MGF inhibited the reactivity of ACC, DGAT2, long-chain acyl-coenzyme A synthase 1 (LACS1), and SCD1 by decreasing SREBP1c and increasing pAMPK [136,137,138]. As a downstream effect of AMPK, changes in ACC should be elaborated more accurately, specifically the pACC to ACC ratio [139]. Moreover, the PPARα pathway was activated following MGF treatment, resulting in increased fatty acid translocase (FAT/CD36), HSL, ATGL, LPL, CPT1 [137, 138]. Consequently, LDs in HepG2 cells and C2C12 cells declined, and FA-induced IR reduced [140, 141]. MGF promotes CPT1-related β-oxidation, and inhibites enzymes related to TAG synthesis to reduce the content of neutral fat nuclei in LDs.
An in vitro model of AS indicated that MGF increased the expression of ATP-binding cassette A1/G1 (ABCA1/G1), PPARγ, and LXRα, promoted macrophage excretion and prevented lipid accumulation [142]. Interestingly, MGF reversed the expression of metabolically active CPT1 in human HT29 colon cells, suggesting that the regulation of fatty acid β-oxidation by MGF varies across models [143]. In addition, the metabolite of MGF, desmethylthiol, reduces the levels of TAG and FA in HepG2 cells by regulating the silent information regulator of transcription 1 (SIRT-1) /AMPK pathway [144]. As regulators of lipid autophagy, PPAR and LXR regulated by MGF increase autophagy and thus decrease the number of LDs.
Capsaicin (CAP)
CAP (Fig. 4C), a phenolic compound derived from the genus Capsicum in the family Solanaceae, is a dietary agent that improves metabolism [145]. In adipocyte experiments, CAP can reduce the size and surface area of LDs by elevating pAMPK, LPL and HSL, PR structural domain containing 16 (PRDM16), CIDEA, PPARγ, uncoupling protein 1 (UCP1) [146,147,148,149,150]. Higher expression of pAMPK also inhibited the AKT/mTOR pathway, decreasing the basal neutrophil content in HepG2 cells [151]. The appliance of CAP in bovine bone marrow mesenchymal stem cells (BMSC) suggests that lipid deposition was inhibited during lipogenic differentiation with a lower transcription of PPARγ, FABP4 and SCD [152, 153].
The in vivo studies have demonstrated that CAP inhibits hepatic LD formation and increases plasma high density lipoprotein-C by activating transient receptor potential vanilloid 1, mediating PPAR, CPT1and CD36, and downregulating fatty acid production (e.g. ACC, FASN), which has implications for the treatment of AS and hypercholesterolemia [154,155,156,157]. It’s also worth noting that CAP cream can be given onto shaved abdominal skin, which provides a new approach for abdominal obesity. CAP mitigates LD accumulation by participating in all processes of lipid metabolism: inhibition of FABP4/SCD-related fatty acid synthesis, promotion of CPT1/CD36-related β-oxidation and lipolysis, and UCP1-mediated adipose tissue browning.
Caffeine (CAF)
CAF (Fig. 4D) is a methylxanthine that appears widely in tea trees and coffee, and clinical studies demonstrated that its consumption has a negative correlation with the level of liver fibrosis [158]. CAF lowers the amount and volume of LDs in over-nourished zebrafish larvae and reduces LD levels in rat adipose-derived stem cells through upregulating CD36, ACOX and downregulating SREBP1c, SREBP2, ACC1, PPARγ, LPL, FASN, UCP2, and SCD1) [159, 160]. The promotion of adipogenic enzymes and inhibition of PPARα induced by HFD are reversed along with higher expression of hepatic CPT1 [161, 162]. CAF inhibits the adipogenic differentiation of fibroblasts by suppressing the transcription of CCAAT/enhancer-binding protein β (C/EBPβ), PPARγ, C/EBPα in Graves, ophthalmopathy [167, 168]. As a whole, ACC1, FASN, and SCD1 are adipogenesis-related proteins, while CD36 and CPT1 are lipogenesis or β-oxidation-related proteins. They are the main targets for CAF to reduce LDs.
In addition, CAF may induce LD autophagy in hepatocytes through rapamycin complex 1 and AMPK, but the exact regulatory mechanism needs further exploration [164]. VELICKOVIC, et al. found that CAF upregulated the browning genes (e.g. UCP1, PPARγ), PRDM16, and PGC-1α in mouse mesenchymal stem cells (MSCs), with LDs becoming smaller and redistributing evenly [163]. MSCs are an in vitro model of BAT, thus the effective concentration for in vivo application requires investigation [164].
Genistein (GEN)
GEN (Fig. 4E), an isoflavone, is the major phytoestrogen in soybean. In a laying-hen FLD model, GEN inhibits the LD formation of hepatocytes [165, 166]. The expression of PPARα and its downstream ACOX, CPT1, and SREBP1c and its downstream GPAT, FASN, LXRα, ACC, and SCD1 is elevated. The expression ofFATP is downregulated [167]. Several in vitro studies also supported the ability of GEN to inhibit FA-induced LD formation in a dose-dependent manner [168].
The PPARγ-SREBP pathway may also play a role in the regulation of LDs by GEN. In the liver of non-obese rats, GEN can enhance SREBP2 and restrain SREBP1, FASN, PPARγ and its target gene CIDEC [169, 170]. It has been reported that GEN downregulate PPARγ and upregulate lipocalin by activation of estrogen receptor β and Akt/mTOR signaling, ameliorating hepatic fat accumulation [171, 172]. The downstream of pathways above requires further elaboration. CIDEC present in LD-mitochondrial contact promotes TAG transfer, while inhibiting the activity of ATGL. Lipid droplet-associated proteins, which undergo opposite alterations upon GEN intervention, are suspected to be due to differences in tissue specificity and induction models. Lipid droplet-associated proteins, which undergo opposite alterations upon GEN intervention, are suspected to be due to differences in tissue specificity and induction models. In conclusion, GEN restricts neutral fat nuclei growth by upregulating ACOX/CPT1-related lipolysis and downregulating ACC/CIDEC/SCD1-related lipogenesis.
Epigallocatechin-3-gallate (EGCG)
EGCG (Fig. 4F), is the major polyphenol and catechin in green tea [173]. EGCG attenuates liver fibrosis, hepatic LDs, glomerular necrosis and FA levels in HFD-fed mice by inhibiting cholesterol synthesis through the SREBP2/SIRT1/FOXO1 pathway [174, 175]. The activation of SREBP2 and its target gene 3-hydroxy-3-methylglutaryl-coenzyme A reductase (HMGCR) enhance cholesterol synthesis [176]. The proteomics indicated a higher expression in FATP1, ACSL1, and CPT2; but a lower expression in SREBP2 and its downstream (ACC1, FASN) [177, 178]. An in vitro study demonstrated that EGCG promoted lysosomal autophagy through a Ca2+/CaMKKβ/AMPK-dependent mechanism in vascular endothelial cells [179].
Moreover, EGCG has tissue variability when coming into effect. EGCG significantly increases the expression of lipid synthesis in WAT while relevant genes in the epithelium tissue are downregulated [180]. In contrast to the control groups, there is a decline in the abdominal lipid rate, LPL, PPARγ and hepatic lipid synthase activity (e.g. FASN, ACC), as well as an increase in ATGL and CPT1 expression [181]. Proteins related to fatty acid transport and oxidation are increased while fatty acid synthesis-related proteins are reduced, resulting in fewer LDs.
Chlorogenic acid (CGA)
CGA (Fig. 4G), a bioactive dietary polyphenol derived from tea and green coffee, can treat hepatic steatosis, cardiovascular diseases, and diabetes [182]. CGA can decrease the amount and density of LDs in liver by upregulating PPARα [183, 184]. Moreover, CGA increases pAMPK to suppress the expression of FASN, PPARγ2 and ACC [185,186,187]. Even so, Mubarak, A. et al. failed to favor the role of CGA in reducing lipid accumulation [188].
A study of diabetic mice showed that CGA had similar effects to metformin in reducing hepatic lipid levels by increasing ATGL, HSL, CPT1a, and ACOX1 and decreasing MGAT1, DGAT1, DGAT2, CD36, FATP4, and LPL) [189, 190]. FATP4 enlarges the core of LDs by mediating. Overall, CGA restricts LD growth by promoting ATGL-associated lipolysis and β-oxidation, and inhibiting of MGAT/DGAT/FATP-related synthesis and transport.
During 3T3-L1 preadipocyte differentiation, CGA downregulates the expression of adipocyte differentiation inhibitor gene and upregulates the expression of adipose transcription factors (e.g. CEBPB, SREBP1), differentiation-related transcription factors (e.g. PPARγ2), and lipogenic pathway-related genes (PLINs, SREBP1) [191]. Therefore, improved adipocyte differentiation changes LDs from spindle-shaped to round, while more HSL reduces intracellular TAG levels [192]. 3T3-L1 preadipocyte undergoes four differentiation stages, thus the effect of CGA in specific stages remains to be clarified [193].
Betaine (BET)
Betaine (Fig. 4H), a trimethyl derivation of glycine, presents in beets, spinach, whole grains, wheat bran [194]. Studies in rats showed that BET diminished the level of LDs and swollen mitochondria in the liver [195]. The expression of DGAT1/2, SREBP1c/2, HSL, ACC and FASN genes is significantly reduced, except for the increased mRNA level of PGC-1α, PGC-1β [196]. In alcohol-induced pancreatic steatosis, BET also reverses pathological changes like LD accumulation and elevated lipocalin levels [197]. However, preclinical models exposed to alcoholic diet cannot summarize hallmarks of human alcohol-associated liver disease (ALD) due to the complicated pathogenesis of ALD [198].
In vitro, smaller LDs deposit uniformly after BET intervention [199]. The genes of SREBP1c, SIRT1, FASN, ACC DGAT2 are downregulated, but genes of MTP, LPL, PPARα, CPT1, ATGL are upregulated [200,201,202]. Similarly, suppression of fatty acid synthesis and deposition, promotion of oxidation and transport by BET maintain LD homeostasis.
Ginsenoside (GIN)
GIN (Fig. 4I), the major active ingredient of Chinese ginseng, contains a group of triterpenoid saponins with different polarity (Rb1, Rg2, Rk3, Rk1, F2, etc.), which apply to IR and FA metabolism [203]. Rb1 promotes CD137, UCP1 [204], PPARα and its downstream (e.g. cholesterol 7 α-hydroxylase, CPT1α/2) in a dosage-dependent approach [205]. The activation of pAMPK (total horizontal of AMPK is unchanged) by Rb1 increases AS plaque stability by inhibiting SREBP, ACC-α, FASN, and SCD1 [206,207,208]. The pathways include activation of autophagic SIRT1/AMPK, PTEN/AKT, and inhibition of extracellular regulated protein kinases (ERK) /p3 and AKT [209, 210]. AKT intervenes the insulin signaling pathway, so natural compounds with insulin-like activities may regulate LDs [211].
In vitro, Rb1 downregulates the expression of FATP2, CD36, FABP1, FATP5, C/EBPβ and transcription factor PPARγ, and upregulates the expression of CPT1 and ACOX1 [212,213,214,215,216]. Siraj, et al. proved that F2 inhibited the mRNA levels of PLINs in adipocytes [217]. As a whole, GIN remarkably reduces LD diameter by promoting CPT1-mediated autophagy and browning-related genes, and inhibiting FA synthesis.
Resveratrol (RES)
RES (Fig. 4J), a dietary polyphenol from red wine and grapes, prevents AS and hepatic steatosis. RES reduces the number of LDs in adipocytes by increasing the expression levels of PGC-1α, UCP1, PRDM16 and CIDEA through the mTOR pathway, AMPK and ACC [218,219,220]. In FA-intervened human L02 hepatocytes, RES can downregulate the expression levels of SREBP1 and its target genes (e.g. SCD1, ACC1, and FAS) [221]. RES reverses the HFD-induced reduction in SIRT1 activity and elevation of—FSP27β/CIDEC, activating transcription factor 6 (ATF6), cyclic-AMP response binding protein H (CREBH), lipophilic, TIP-7 especially PLINs) [222,223,224]. Similarly, additional study found that RES could alleviate the expression of the adipose differentiation-related protein in the mouse liver through SIRT1/ATF6 signaling pathway [225]. SIRT1/AMPK also regulates mitochondrial autophagy [226, 227]. As a sensor of energy metabolism, SIRT1 regulates over 70 substrates like PGC-1α and Forkhead Box O (FOXO) by sensing the changes of Nicotinamide Adenine Dinucleotide (NAD+) levels [228]. Therefore, RES reduces LD accumulation by inhibiting FA synthesis and promoting adipose tissue browning.
A study on obesity found that RES attenuated the expression level of PLIN5 in BAT and heart tissue [229], while increasing the expression of PLIN5 in skeletal muscle. The clinical trial also manifested that the number of LDs in myocytes from T2DM patients increased after RES intake and LDs containing PLIN5 increased notably, but this change may help mitigate IR [230]. As a potential direct target of RES in the LD-mitochondrial contact, PLIN5 interacts with FATP, facilitating FA transfer.
Discussion
In recent years, LDs has been acknowledged as an organelle whose size, number, morphology, and composition vary dynamically in response to an individual’s metabolic state. From a physiological standpoint, LD biogenesis serves to sequester detrimental free FAs, thereby mitigating ER stress and oxidative stress induced by lipotoxicity. Disruption of LD homeostasis can result in impaired or overloaded fatty acid storage, leading to lipotoxicity-related diseases like T2DM and fatty liver. Excessive accumulation or ectopic deposition of LDs is also closely related to the pathology of cardiovascular disease, chronic kidney disease, etc. To summarize, the significant role of LDs in chronic diseases associated with lipid metabolism has been confirmed. At the same time, LD-associated proteins have also gradually attracted significant attention. This class of proteins participants in LD biosynthesis and is also the direct executor of their biological functions. Targeting LD-associated proteins to regulate the “fate” of LDs may hold great promise for the development of innovative therapies. Given that LDs exhibit dynamic changes, their state varies in different diseases or at different stages of the same disease. As such, it is crucial to flexibly regulate LD homeostasis and biological functions.
Drugs targeting LD-associated proteins are already in clinical use. DGAT2 inhibitors and ACC inhibitors can reduce liver fibrosis [231]. ACAT1 inhibitors disrupt the biogenesis of CE-rich LDs, reducing cancer proliferation and aggressiveness in prostate cancer [232]. Nonetheless, these specific inhibitors have the potential to cause harm to other regular cellular metabolic processes [54].
Natural compounds have positive prospects in the treatment of metabolic diseases due to their safety, effectiveness and multiple targets. There are numerous natural compounds with lipid-regulating, glucose-lowering, and oxidative stress-reducing effects. However, their pharmacological mechanisms are not been fully understood, limiting their potential for clinical use. We outlined the mechanism of 10 common natural compounds, focusing on the LD-associated proteins and pathways they regulate. We emphasize that LDs may be a target organelle of natural compounds, and LD-associated proteins may serve as important targets of these compounds or downstream effectors or upstream regulators of pathways (Fig. 5).
Despite their potential, research on natural compounds related to LD-associated proteins remains restricted:
-
(1)
Most studies are in vivo and in vitro studies, with a lack of clinical data.
-
(2)
Only phenotypic improvement and regulatory pathway data are presented, without in-depth analyses like protein interaction.
-
(3)
Limited focus is placed on LD-associated proteins, concentrating on PLINs and CIDEs.
-
(4)
Attention is mainly on LD in the liver or adipose, with less focus on cardiovascular, cerebrovascular, kidney and other organs.
Future studies should employ drug-target identification methods to focus more closely on the direct targets of natural compounds, specifically LD-associated proteins. The specific effects of natural compounds on LD-associated proteins, including post-translational modifications and protein interactions, remain unclear. As LD is a dynamically changing organelle, it is essential to understand how natural compounds regulate LD biology in different diseases or stages of the same disease. Frequent contact between LDs and other organelles can affect LD homeostasis, so it is important to investigate whether natural compounds mediate this contact.
Natural compounds also face challenges such as rapid metabolism, insufficient absorption, and poor solubility. For example, CAP has a significant first pass metabolism and a very short half-life by intravenous administration [233, 234]; BBR has poor solubility with less than 1% of oral bioavailability [235]. These drawbacks result in limitations in clinical use and dissemination [236]. As a result, the development of metabolites or derivatives from natural compounds with higher bioavailability may facilitate their clinical applications.
Conclusion
This review has summarized the current understanding of LDs, LD-associated proteins, and pathological changes arising from abnormal LD metabolism. Moreover, we provide the first overview of the therapeutic mechanisms of natural compounds with lipid-modulating effects in metabolic diseases from the perspective of LDs and LD-associated proteins.
Previous research has shown that natural compounds positively impact metabolic diseases by regulating LD homeostasis. The underlying mechanisms may be closely related to biological processes such as LD biogenesis, growth, fusion, and degradation mediated by LD-associated proteins including PLINs, CIDEs, neutral lipid synthases, lipolytic enzymes, and so on. LD deserves extensive attention as a target organelle for natural compounds. Given that natural compounds exhibit a wide range of biological activities, their therapeutic effects may also involve multiple signaling pathways. This complexity makes it difficult to elucidate whether LD-associated proteins can be direct targets of action for natural compounds with the available evidence. As a novel research target for this class of drugs, there is a need to accurately identify more LD-associated proteins, and provide more reliable and direct evidence of drug-target interactions. Challenges remain to improve the bioavailability of natural compounds and to conduct relevant clinical trials. All in all, future studies on LD and LD-associated proteins will hold promising prospects for the development and utilization of natural compounds.
Availability of data and materials
Not applicable.
Abbreviations
- LD:
-
Lipid droplet
- NAFLD:
-
Non-alcoholic fatty liver disease
- PLIN:
-
Perilipin
- CIDE:
-
Cell death-induced DNA fragmentation factor 45-like effector
- HIG2:
-
Hypoxia inducible gene 2
- TAG:
-
Triacylglycerol
- CE:
-
Cholesterol ester
- FA:
-
Fatty acid
- DAG:
-
Diacylglycerol
- ER:
-
Endoplasmic reticulum
- LDAF1:
-
LD assembly factor 1
- AGPAT2:
-
1-Acylglycerol-3-phosphate O-acyltransferase 2
- Lipin-1:
-
Lipoprotein 1
- PA:
-
Palmitic acid
- GPAT:
-
Glycerol-3-phosphate acyltransferase
- nLD:
-
Nucleus LD
- cLD:
-
Cytoplasmic LD
- PC:
-
Phosphatidylcholine
- PCYT:
-
Phosphocholine cytidylyltransferase
- DGAT2:
-
Diacylglycerol acyltransferase 2
- PAP:
-
Phosphatidic acid phosphatase
- MFN2:
-
Mitofusin2
- HSC70:
-
Heat shock cognate 71 kDa protein
- ARFRP1:
-
ADP-ribosylation factor related protein 1
- SNAP23:
-
Synaptosome-associated protein 23
- MIGA2:
-
Mitoguardin 2
- VPS13D:
-
Vacuolar Protein Sorting 13D
- UBXD8:
-
UBX structural domain-containing protein 8
- CGI-58:
-
Comparative gene identification-58
- ATGL:
-
Adipose triglyceride lipase
- PNPLA2:
-
Patatin-like phospholipase domain-containing protein 2
- WAT:
-
White adipose tissue
- HSL:
-
Hormone-sensitive triglyceride lipase
- MAGL:
-
Monoacylglycerol lipase
- SREBP:
-
Sterol regulatory element binding protein
- LXR:
-
Liver X receptor
- PPAR:
-
Peroxisome proliferators-activated receptor
- CMA:
-
Chaperone-mediated autophagy
- HOPS:
-
Homotypic fusion and protein sorting
- SNARE:
-
Soluble NSF attachment protein receptor
- FASN:
-
Fatty acid synthase
- HFD:
-
High-fat-diet
- AMPK:
-
Adenosine 5'-monophosphate-activated protein kinase
- VLDL:
-
Very low-density lipoprotein
- T2DM:
-
Type 2 diabetes mellitus
- 1-MAG:
-
1-Monoacylglycerols
- STX1a:
-
Synthesis protein 1a
- CKD:
-
Chronic kidney disease
- CPT1:
-
Carnitine palmitoyltransferases 1
- ACOX1:
-
Acyl-Coenzyme A oxidase 1
- L-FABP:
-
L-fatty acid binding protein
- LDLR:
-
LDL receptor
- DN:
-
Diabetic nephropathy
- AKI:
-
Acute kidney injury
- PGC-1α:
-
PPARγ coactivator-1α
- AS:
-
Atherosclerosis
- ACAT1:
-
Acyl coenzyme A-cholesterol acyltransferase
- IRI:
-
Ischemia–reperfusion injury
- ACSL:
-
Acyl-CoA synthetase long chain family member
- CGI:
-
Congenital lipodystrophy
- IR:
-
Insulin resistance
- mTOR:
-
Mammalian target of rapamycin;
- BBR:
-
Berberine;
- SCD:
-
Stearoyl coenzyme a desaturase
- ACCα:
-
Acetyl coenzyme carboxylase α
- LPL:
-
Lipoprotein lipase
- MGF:
-
Mangostin
- LACS1:
-
Long-chain acyl-coenzyme A synthase 1
- ABCA1/G1:
-
ATP-binding cassette A1/G1
- SIRT1:
-
Silent information regulator of transcription 1
- PRDM16:
-
PR structural domain containing 16
- UCP1:
-
Uncoupling protein 1
- BMSC:
-
Bone marrow mesenchymal stem cell
- MSCs:
-
Mesenchymal stem cells
- C/EBPβ:
-
CCAAT/enhancer-binding protein β
- GEN:
-
Genistein
- FATP:
-
Fatty acid transporter protein
- EGCG:
-
Epigallocatechin-3-gallate
- HMGCR:
-
3-Hydroxy-3-methylglutaryl-coenzyme A reductase
- CGA:
-
Chlorogenic acid
- ALD:
-
Alcohol-associated liver disease
- ERK:
-
Extracellular regulated protein kinases
- RES:
-
Resveratrol
- ATF:
-
Activating transcription factor
- CREBH:
-
Cyclic-AMP response binding protein H
- FOXO:
-
Forkhead Box O
- NAD+ :
-
Nicotinamide adenine dinucleotide
- CAP:
-
Capsaicin
- CAF:
-
Caffeine
- BET:
-
Betaine
- GIN:
-
Ginsenoside
Reference:s
Li D, Li Y, Yang S, Lu J, Jin X, Wu M. Diet-gut microbiota-epigenetics in metabolic diseases: From mechanisms to therapeutics. Biomed Pharmacother. 2022;153:113290.
Boutari C, Mantzoros CS. A 2022 update on the epidemiology of obesity and a call to action: as its twin COVID-19 pandemic appears to be receding, the obesity and dysmetabolism pandemic continues to rage on. Metabolism. 2022;133: 155217.
Riazi K, Swain MG, Congly SE, Kaplan GG, Shaheen AA. Race and ethnicity in non-alcoholic fatty liver disease (NAFLD): a narrative review. Nutrients. 2022;14:21.
Alferink LJM, Erler NS, de Knegt RJ, Janssen HLA, Metselaar HJ, Darwish Murad S, et al. Adherence to a plant-based, high-fibre dietary pattern is related to regression of non-alcoholic fatty liver disease in an elderly population. Eur J Epidemiol. 2020;35(11):1069–85.
Fujimoto T. Lipid droplet as an independent organelle. Seikagaku. 2004;76(6):578–84.
Dhiman R, Caesar S, Thiam AR, Schrul B. Mechanisms of protein targeting to lipid droplets: a unified cell biological and biophysical perspective. Semin Cell Dev Biol. 2020;108:4–13.
Yamaguchi T. PAT family: lipid droplet-associated proteins that regulate fat storage and lipolysis. Seikagaku. 2007;79(2):162–6.
Chang BH, Chan L. Regulation of triglyceride metabolism. III. Emerging role of lipid droplet protein ADFP in health and disease. Am J Physiol Gastrointest Liver Physiol. 2007;292(6):G1465-8.
Brasaemle DL. Thematic review series: adipocyte biology. The perilipin family of structural lipid droplet proteins stabilization of lipid droplets and control of lipolysis. J Lipid Res. 2007;48(12):2547–59.
Liu MF, Xu GH. Function of PAT family proteins in the lipid metabolism. Sheng Li Ke Xue Jin Zhan. 2006;37(2):103–7.
Gao G, Chen FJ, Zhou L, Su L, Xu D, Xu L, et al. Control of lipid droplet fusion and growth by CIDE family proteins. Biochim Biophys Acta Mol Cell Biol Lipids. 2017;1862:1197–204.
Gimm T, Wiese M, Teschemacher B, Deggerich A, Schödel J, Knaup KX, et al. Hypoxia-inducible protein 2 is a novel lipid droplet protein and a specific target gene of hypoxia-inducible factor-1. Faseb j. 2010;24(11):4443–58.
Seebacher F, Zeigerer A, Kory N, Krahmer N. Hepatic lipid droplet homeostasis and fatty liver disease. Semin Cell Dev Biol. 2020;108:72–81.
Chen FJ, Yin Y, Chua BT, Li P. CIDE family proteins control lipid homeostasis and the development of metabolic diseases. Traffic. 2020;21(1):94–105.
Walther TC, Chung J, Farese RV Jr. Lipid droplet biogenesis. Annu Rev Cell Dev Biol. 2017;33:491–510.
Olzmann JA, Carvalho P. Dynamics and functions of lipid droplets. Nat Rev Mol Cell Biol. 2019;20(3):137–55.
Li Z, Johnson MR, Ke Z, Chen L, Welte MA. Drosophila lipid droplets buffer the H2Av supply to protect early embryonic development. Current Biol. 2014;24:13.
Olarte M-J, Swanson JMJ, Walther TC, Farese RV Jr. The CYTOLD and ERTOLD pathways for lipid droplet-protein targeting. Trends Biochem Sci. 2022;47(1):39–51.
Henne M, Goodman JM, Hariri H. Spatial compartmentalization of lipid droplet biogenesis. Biochim Biophys Acta Mol Cell Biol Lipids. 2020;1865(1): 158499.
Zhang C, Liu P. The new face of the lipid droplet: lipid droplet proteins. Proteomics. 2019;19(10): e1700223.
Olarte MJ, Swanson JMJ, Walther TC, Farese RV Jr. The CYTOLD and ERTOLD pathways for lipid droplet-protein targeting. Trends Biochem Sci. 2022;47(1):39–51.
Roberts MA, Olzmann JA. Protein quality control and lipid droplet metabolism. Annu Rev Cell Dev Biol. 2020;36:115–39.
D’Aquila T, Sirohi D, Grabowski JM, Hedrick VE, Paul LN, Greenberg AS, et al. Characterization of the proteome of cytoplasmic lipid droplets in mouse enterocytes after a dietary fat challenge. PLoS ONE. 2015;10(5): e0126823.
Smirnova E, Goldberg EB, Makarova KS, Lin L, Brown WJ, Jackson CL. ATGL has a key role in lipid droplet/adiposome degradation in mammalian cells. EMBO Rep. 2006;7(1):106–13.
Moessinger C, Kuerschner L, Spandl J, Shevchenko A, Thiele C. Human lysophosphatidylcholine acyltransferases 1 and 2 are located in lipid droplets where they catalyze the formation of phosphatidylcholine. J Biol Chem. 2011;286(24):21330–9.
Kory N, Grond S, Kamat SS, Li Z, Krahmer N, Chitraju C, et al. Mice lacking lipid droplet-associated hydrolase, a gene linked to human prostate cancer, have normal cholesterol ester metabolism. J Lipid Res. 2017;58(1):226–35.
Castro IG, Eisenberg-Bord M, Persiani E, Rochford JJ, Schuldiner M, Bohnert M. Promethin is a conserved seipin partner protein. Cells. 2019;8:3.
Suzuki M, Otsuka T, Ohsaki Y, Cheng J, Taniguchi T, Hashimoto H, et al. Derlin-1 and UBXD8 are engaged in dislocation and degradation of lipidated ApoB-100 at lipid droplets. Mol Biol Cell. 2012;23(5):800–10.
Saka HA, Thompson JW, Chen YS, Dubois LG, Haas JT, Moseley A, et al. Chlamydia trachomatis infection leads to defined alterations to the lipid droplet proteome in epithelial cells. PLoS ONE. 2015;10(4): e0124630.
Čopič A, Antoine-Bally S, Giménez-Andrés M, La Torre GC, Antonny B, Manni MM, et al. A giant amphipathic helix from a perilipin that is adapted for coating lipid droplets. Nat Commun. 2018;9(1):1332.
Krahmer N, Guo Y, Wilfling F, Hilger M, Lingrell S, Heger K, et al. Phosphatidylcholine synthesis for lipid droplet expansion is mediated by localized activation of CTP:phosphocholine cytidylyltransferase. Cell Metab. 2011;14(4):504–15.
Itabe H, Yamaguchi T, Nimura S, Sasabe N. Perilipins: a diversity of intracellular lipid droplet proteins. Lipids Health Dis. 2017;16(1):83.
Filali-Mouncef Y, Hunter C, Roccio F, Zagkou S, Dupont N, Primard C, et al. The ménage à trois of autophagy, lipid droplets and liver disease. Autophagy. 2022;18(1):50–72.
Olzmann JA-O, Carvalho PA-O. Dynamics and functions of lipid droplets. Nat Rev Mol Cell Biol. 2019;20:3.
Cao Z, Wang X, Huang X, Mak HY. Are endoplasmic reticulum subdomains shaped by asymmetric distribution of phospholipids? Evidence from a C elegans model system. Bioessays. 2021;43(1):e2000199.
Chung J, Wu X, Lambert TJ, Lai ZW, Walther TC, Farese RV Jr. LDAF1 and Seipin Form a Lipid Droplet Assembly Complex. Dev Cell. 2019;51(5):551-63.e7.
Rao MJ, Goodman JM. Seipin: harvesting fat and keeping adipocytes healthy. Trends Cell Biol. 2021;31(11):912–23.
Zoni V, Khaddaj R, Lukmantara I, Shinoda W, Yang H, Schneiter R, et al. Seipin accumulates and traps diacylglycerols and triglycerides in its ring-like structure. Proc Natl Acad Sci U S A. 2021;118:10.
Talukder MM, Sim MF, O’Rahilly S, Edwardson JM, Rochford JJ. Seipin oligomers can interact directly with AGPAT2 and lipin 1, physically scaffolding critical regulators of adipogenesis. Mol Metab. 2015;4(3):199–209.
Pagac M, Cooper DE, Qi Y, Lukmantara IE, Mak HY, Wu Z, et al. SEIPIN regulates lipid droplet expansion and adipocyte development by modulating the activity of glycerol-3-phosphate acyltransferase. Cell Rep. 2016;17(6):1546–59.
Layerenza JP, González P, García de Bravo MM, Polo MP, Sisti MS, Ves-Losada A. Nuclear lipid droplets: a novel nuclear domain. Biochim Biophys Acta. 2013;1831(2):327–40.
Wang L, Wang Y, Liang Y, Li J, Liu Y, Zhang J, et al. Specific accumulation of lipid droplets in hepatocyte nuclei of PFOA-exposed BALB/c mice. Sci Rep. 2013;3:2174.
Wolinski H, Hofbauer HF, Hellauer K, Cristobal-Sarramian A, Kolb D, Radulovic M, et al. Seipin is involved in the regulation of phosphatidic acid metabolism at a subdomain of the nuclear envelope in yeast. Biochim Biophys Acta. 2015;1851(11):1450–64.
Barneda D, Christian M. Lipid droplet growth: regulation of a dynamic organelle. Curr Opin Cell Biol. 2017;47:9–15.
Haider A, Wei YC, Lim K, Barbosa AD, Liu CH, Weber U, et al. PCYT1A regulates phosphatidylcholine homeostasis from the inner nuclear membrane in response to membrane stored curvature elastic stress. Dev Cell. 2018;45(4):481-95.e8.
Lee J, Ridgway ND. Phosphatidylcholine synthesis regulates triglyceride storage and chylomicron secretion by Caco2 cells. J Lipid Res. 2018;59(10):1940–50.
Gong J, Sun Z, Wu L, Xu W, Schieber N, Xu D, et al. Fsp27 promotes lipid droplet growth by lipid exchange and transfer at lipid droplet contact sites. J Cell Biol. 2011;195(6):953–63.
Jambunathan S, Yin J, Khan W, Tamori Y, Puri V. FSP27 promotes lipid droplet clustering and then fusion to regulate triglyceride accumulation. PLoS ONE. 2011;6(12): e28614.
Xu W, Wu L, Yu M, Chen FJ, Arshad M, Xia X, et al. Differential roles of cell death-inducing dna fragmentation factor-α-like effector (CIDE) proteins in promoting lipid droplet fusion and growth in subpopulations of hepatocytes. J Biol Chem. 2016;291(9):4282–93.
Wilfling F, Wang H, Haas JT, Krahmer N, Gould TJ, Uchida A, et al. Triacylglycerol synthesis enzymes mediate lipid droplet growth by relocalizing from the ER to lipid droplets. Dev Cell. 2013;24(4):384–99.
Valdearcos M, Esquinas E, Meana C, Gil-de-Gómez L, Guijas C, Balsinde J, et al. Subcellular localization and role of lipin-1 in human macrophages. J Immunol. 2011;186(10):6004–13.
Ohsaki Y, Sołtysik K, Fujimoto T. The lipid droplet and the endoplasmic reticulum. Adv Exp Med Biol. 2017;997:111–20.
Huang W, Gao F, Zhang Y, Chen T, Xu C. Lipid droplet-associated proteins in cardiomyopathy. Ann Nutr Metab. 2022;78(1):1–13.
Zadoorian A, Du X, Yang H. Lipid droplet biogenesis and functions in health and disease. Nat Rev Endocrinol. 2023;19(8):443–59.
Prinz WA. Bridging the gap: membrane contact sites in signaling, metabolism, and organelle dynamics. J Cell Biol. 2014;205(6):759–69.
Herms A, Bosch M, Reddy BJ, Schieber NL, Fajardo A, Rupérez C, et al. AMPK activation promotes lipid droplet dispersion on detyrosinated microtubules to increase mitochondrial fatty acid oxidation. Nat Commun. 2015;6:7176.
Granneman JG, Moore HP, Mottillo EP, Zhu Z, Zhou L. Interactions of perilipin-5 (Plin5) with adipose triglyceride lipase. J Biol Chem. 2011;286(7):5126–35.
Boutant M, Kulkarni SS, Joffraud M, Ratajczak J, Valera-Alberni M, Combe R, et al. Mfn2 is critical for brown adipose tissue thermogenic function. Embo j. 2017;36(11):1543–58.
Fan H, Tan Y. Lipid droplet-mitochondria contacts in health and disease. Int J Mol Sci. 2024;25:13.
Dirkx R, Vanhorebeek I, Martens K, Schad A, Grabenbauer M, Fahimi D, et al. Absence of peroxisomes in mouse hepatocytes causes mitochondrial and ER abnormalities. Hepatology. 2005;41(4):868–78.
Binns D, Januszewski T, Chen Y, Hill J, Markin VS, Zhao Y, et al. An intimate collaboration between peroxisomes and lipid bodies. J Cell Biol. 2006;173(5):719–31.
Young SG, Zechner R. Biochemistry and pathophysiology of intravascular and intracellular lipolysis. Genes Dev. 2013;27(5):459–84.
Zimmermann R, Lass A, Haemmerle G, Zechner R. Fate of fat: the role of adipose triglyceride lipase in lipolysis. Biochim Biophys Acta. 2009;1791(6):494–500.
Grabner GF, Zimmermann R, Schicho R, Taschler U. Monoglyceride lipase as a drug target: at the crossroads of arachidonic acid metabolism and endocannabinoid signaling. Pharmacol Ther. 2017;175:35–46.
Zechner R, Madeo F, Kratky D. Cytosolic lipolysis and lipophagy: two sides of the same coin. Nat Rev Mol Cell Biol. 2017;18(11):671–84.
Kimmel AR, Sztalryd C. The perilipins: major cytosolic lipid droplet-associated proteins and their roles in cellular lipid storage, mobilization, and systemic homeostasis. Annu Rev Nutr. 2016;36:471–509.
Gutierrez MG, Munafó DB, Berón W, Colombo MI. Rab7 is required for the normal progression of the autophagic pathway in mammalian cells. J Cell Sci. 2004;117(Pt 13):2687–97.
Balderhaar HJ, Ungermann C. CORVET and HOPS tethering complexes - coordinators of endosome and lysosome fusion. J Cell Sci. 2013;126(Pt 6):1307–16.
Jiang JJ, Zhang GF, Zheng JY, Sun JH, Ding SB. Targeting mitochondrial ROS-mediated ferroptosis by quercetin alleviates high-fat diet-induced hepatic lipotoxicity. Front Pharmacol. 2022;13: 876550.
Willebrords J, Pereira IV, Maes M, Crespo Yanguas S, Colle I, Van Den Bossche B, et al. Strategies, models and biomarkers in experimental non-alcoholic fatty liver disease research. Prog Lipid Res. 2015;59:106–25.
Mastoridou EM, Goussia AC, Kanavaros P, Charchanti AV. Involvement of lipophagy and chaperone-mediated autophagy in the pathogenesis of non-alcoholic fatty liver disease by regulation of lipid droplets. Int J Mol Sci. 2023;24:21.
Straub BK. Lipid droplet-associated proteins. Importance in steatosis, steatohepatitis and hepatocarcinogenesis. Der Pathologe. 2015. https://doiorg.publicaciones.saludcastillayleon.es/10.1007/s00292-015-0082-3.
Sharma D, Mandal P. NAFLD: genetics and its clinical implications. Clin Res Hepatol Gastroenterol. 2022;46(9): 102003.
Langhi C, Arias N, Rajamoorthi A, Basta J, Lee RG, Baldán Á. Therapeutic silencing of fat-specific protein 27 improves glycemic control in mouse models of obesity and insulin resistance. J Lipid Res. 2017;58(1):81–91.
McVicker BL, Rasineni K, Tuma DJ, McNiven MA, Casey CA. Lipid droplet accumulation and impaired fat efflux in polarized hepatic cells: consequences of ethanol metabolism. Int J Hepatol. 2012;2012: 978136.
Sans A, Bonnafous S, Rousseau D, Patouraux S, Canivet CM, Leclere PS, et al. The differential expression of cide family members is associated with Nafld progression from steatosis to steatohepatitis. Sci Rep. 2019;9(1):7501.
Listenberger LL, Ostermeyer-Fay AG, Goldberg EB, Brown WJ, Brown DA. Adipocyte differentiation-related protein reduces the lipid droplet association of adipose triglyceride lipase and slows triacylglycerol turnover. J Lipid Res. 2007;48(12):2751–61.
Ma YY, Yin XC, Qin ZZ, Ke XF, Mi Y, Zheng PY, et al. Role of Plin5 deficiency in progression of non-alcoholic fatty liver disease induced by a high-fat diet in mice. J Comp Pathol. 2021;189:88–97.
Radner FP, Streith IE, Schoiswohl G, Schweiger M, Kumari M, Eichmann TO, Rechberger, et al. Growth retardation, impaired triacylglycerol catabolism, hepatic steatosis, and lethal skin barrier defect in mice lacking comparative gene identification-58 CGI-58. J Biol Chem. 2010;285:10.
Wang Y, Kory N, BasuRay S, Cohen JC, Hobbs HH. PNPLA3, CGI-58, and inhibition of hepatic triglyceride hydrolysis in mice. Hepatology. 2019;69:6.
Rasineni K, McVicker BL, Tuma DJ, McNiven MA, Casey CA. Rab GTPases associate with isolated lipid droplets (LDs) and show altered content after ethanol administration: potential role in alcohol-impaired LD metabolism. Alcohol Clin Exp Res. 2014;38(2):327–35.
Ye J, Li JZ, Liu Y, Li X, Yang T, Ma X, et al. Cideb, an ER- and lipid droplet-associated protein, mediates VLDL lipidation and maturation by interacting with apolipoprotein B. Cell Metab. 2009;9(2):177–90.
Dai C, Kayton NS, Shostak A, Poffenberger G, Cyphert HA, Aramandla R, et al. Stress-impaired transcription factor expression and insulin secretion in transplanted human islets. J Clin Invest. 2016;126(5):1857–70.
Li Q, Zhao Y, Guo H, Li Q, Yan C, Li Y, et al. Impaired lipophagy induced-microglial lipid droplets accumulation contributes to the buildup of TREM1 in diabetes-associated cognitive impairment. Autophagy. 2023;19(10):2639–56.
Yang M, Luo S, Yang J, Chen W, He L, Liu D, et al. Lipid droplet - mitochondria coupling: A novel lipid metabolism regulatory hub in diabetic nephropathy. Front Endocrinol (Lausanne). 2022;13:1017387.
Schelling JR. The contribution of lipotoxicity to diabetic kidney disease. Cells. 2022;11:20.
Yoshioka K, Hirakawa Y, Kurano M, Ube Y, Ono Y, Kojima K, et al. Lysophosphatidylcholine mediates fast decline in kidney function in diabetic kidney disease. Kidney Int. 2022;101(3):510–26.
Tang T, Abbott MJ, Ahmadian M, Lopes AB, Wang Y, Sul HS. Desnutrin/ATGL activates PPARδ to promote mitochondrial function for insulin secretion in islet β cells. Cell Metab. 2013;18(6):883–95.
Zhao S, Mugabo Y, Iglesias J, Xie L, Delghingaro-Augusto V, Lussier R, et al. α/β-Hydrolase domain-6-accessible monoacylglycerol controls glucose-stimulated insulin secretion. Cell Metab. 2014;19(6):993–1007.
Liu S, Promes JA, Harata M, Mishra A, Stephens SB, Taylor EB, et al. Adipose triglyceride lipase is a key lipase for the mobilization of lipid droplets in human β-cells and critical for the maintenance of syntaxin 1a levels in β-cells. Diabetes. 2020;69(6):1178–92.
Ma H, Guo X, Cui S, Wu Y, Zhang Y, Shen X, et al. Dephosphorylation of AMP-activated protein kinase exacerbates ischemia/reperfusion-induced acute kidney injury via mitochondrial dysfunction. Kidney Int. 2022;101(2):315–30.
den Braanker DJW, Maas RJH, van Mierlo G, Parr NMJ, Bakker-van Bebber M, Deegens JKJ, et al. Primary focal segmental glomerulosclerosis plasmas increase lipid droplet formation and perilipin-2 expression in human podocytes. Int J Mol Sci. 2022;24:1.
Li H, Dixon EE, Wu H, Humphreys BD. Comprehensive single-cell transcriptional profiling defines shared and unique epithelial injury responses during kidney fibrosis. Cell Metab. 2022;34(12):1977-98.e9.
Chen Y, Dai Y, Song K, Huang Y, Zhang L, Zhang C, et al. Pre-emptive pharmacological inhibition of fatty acid-binding protein 4 attenuates kidney fibrosis by reprogramming tubular lipid metabolism. Cell Death Dis. 2021;12(6):572.
Vijayan A. Tackling AKI: prevention, timing of dialysis and follow-up. Nat Rev Nephrol. 2021;17(2):87–8.
Yuan Y, Sun H, Sun Z. Advanced glycation end products (AGEs) increase renal lipid accumulation: a pathogenic factor of diabetic nephropathy (DN). Lipids Health Dis. 2017;16(1):126.
Yu M, Wang H, Zhao J, Yuan Y, Wang C, Li J, et al. Expression of CIDE proteins in clear cell renal cell carcinoma and their prognostic significance. Mol Cell Biochem. 2013;378(1–2):145–51.
Chen Z, Shrestha R, Yang X, Wu X, Jia J, Chiba H, et al. Oxidative stress and lipid dysregulation in lipid droplets: a connection to chronic kidney disease revealed in human kidney cells. Antioxidants (Basel). 2022;11:7.
Mitrofanova A, Merscher S, Fornoni A. Kidney lipid dysmetabolism and lipid droplet accumulation in chronic kidney disease. Nat Rev Nephrol. 2023;19(10):629–45.
Chen FL, Yang ZH, Wang XC, Liu Y, Yang YH, Li LX, et al. Adipophilin affects the expression of TNF-alpha, MCP-1, and IL-6 in THP-1 macrophages. Mol Cell Biochem. 2010;337(1–2):193–9.
Nuotio K, Isoviita PM, Saksi J, Ijäs P, Pitkäniemi J, Sonninen R, et al. Adipophilin expression is increased in symptomatic carotid atherosclerosis: correlation with red blood cells and cholesterol crystals. Stroke. 2007;38(6):1791–8.
Larigauderie G, Furman C, Jaye M, Lasselin C, Copin C, Fruchart JC, et al. Adipophilin enhances lipid accumulation and prevents lipid efflux from THP-1 macrophages: potential role in atherogenesis. Arterioscler Thromb Vasc Biol. 2004;24(3):504–10.
Li JZ, Lei Y, Wang Y, Zhang Y, Ye J, Xia X, et al. Control of cholesterol biosynthesis, uptake and storage in hepatocytes by Cideb. Biochim Biophys Acta. 2010;1801(5):577–86.
Goo YH, Son SH, Kreienberg PB, Paul A. Novel lipid droplet-associated serine hydrolase regulates macrophage cholesterol mobilization. Arterioscler Thromb Vasc Biol. 2014;34(2):386–96.
Ethem İ, Hacıoğlu C. Effects of perilipin-5 on lipid metabolism and high-sensitivity cardiac troponin I. Rev Assoc Med Bras. 2022;68(8):1011–6.
Zhao YB, Zhao J, Zhang LJ, Shan RG, Sun ZZ, Wang K, et al. MicroRNA-370 protects against myocardial ischemia/reperfusion injury in mice following sevoflurane anesthetic preconditioning through PLIN5-dependent PPAR signaling pathway. Biomed Pharmacother. 2019;113: 108697.
Nakamura M, Sadoshima J. Cardiomyopathy in obesity, insulin resistance and diabetes. J Physiol. 2020;598(14):2977–93.
Currie E, Schulze A, Zechner R, Walther TC, Farese RV Jr. Cellular fatty acid metabolism and cancer. Cell Metab. 2013;18(2):153–61.
Weinberg JM. Lipotoxicity. Kidney Int. 2006;70(9):1560–6.
Chen JR, Ding CF, Chen YH, Hu WD, Yu CK, Peng CH, et al. ACSL4 reprograms fatty acid metabolism in hepatocellular carcinoma via c-Myc/SREBP1 pathway. Cancer Lett. 2021;502:154–65.
Quan Y, Dai J, Zhou S, Zhao L, Jin L, Long Y, et al. HIF2α-induced upregulation of RNASET2 promotes triglyceride synthesis and enhances cell migration in clear cell renal cell carcinoma. FEBS Open Bio. 2023. https://doiorg.publicaciones.saludcastillayleon.es/10.1002/2211-5463.13570.
Klasson TD, LaGory EL, Zhao H, Huynh SK, Papandreou I, Moon EJ, et al. ACSL3 regulates lipid droplet biogenesis and ferroptosis sensitivity in clear cell renal cell carcinoma. Cancer Metab. 2022;10(1):14.
Wang K, Ruan H, Song Z, Cao Q, Bao L, Liu D, et al. PLIN3 is up-regulated and correlates with poor prognosis in clear cell renal cell carcinoma. Urol Oncol. 2018;36:7.
Moldavski O, Amen T, Levin-Zaidman S, Eisenstein M, Rogachev I, Brandis A, et al. Lipid droplets are essential for efficient clearance of cytosolic inclusion bodies. Dev Cell. 2015;33(5):603–10.
Luo W, Wang H, Ren L, Lu Z, Zheng Q, Ding L, et al. Adding fuel to the fire: the lipid droplet and its associated proteins in cancer progression. Int J Biol Sci. 2022;18(16):6020–34.
Morrissey JJ, Mobley J, Figenshau RS, Vetter J, Bhayani S, Kharasch ED. Urine aquaporin 1 and perilipin 2 differentiate renal carcinomas from other imaged renal masses and bladder and prostate cancer. Mayo Clin Proc. 2015;90(1):35–42.
Lucenay KS, Doostan I, Karakas C, Bui T, Ding Z, Mills GB, et al. Cyclin E associates with the lipogenic enzyme ATP-citrate lyase to enable malignant growth of breast cancer cells. Cancer Res. 2016;76(8):2406–18.
Matsubara J, Honda K, Ono M, Sekine S, Tanaka Y, Kobayashi M, et al. Identification of adipophilin as a potential plasma biomarker for colorectal cancer using label-free quantitative mass spectrometry and protein microarray. Cancer Epidemiol Biomarkers Prev. 2011;20(10):2195–203.
Zhang X, Su L, Sun K. Expression status and prognostic value of the perilipin family of genes in breast cancer. Am J Transl Res. 2021;13(5):4450–63.
Zammouri J, Vatier C, Capel E, Auclair M, Storey-London C, Bismuth E, et al. Molecular and cellular bases of lipodystrophy syndromes. Front Endocrinol (Lausanne). 2021;12: 803189.
Xu S, Zhang X, Liu P. Lipid droplet proteins and metabolic diseases. Biochim Biophys Acta Mol Basis Dis. 2018. https://doiorg.publicaciones.saludcastillayleon.es/10.1016/j.bbadis.2017.07.019.
Moriya K, Yotsuyanagi H, Shintani Y, Fujie H, Ishibashi K, Matsuura Y, et al. Hepatitis C virus core protein induces hepatic steatosis in transgenic mice. J Gen Virol. 1997;78(Pt 7):1527–31.
Salloum S, Wang H, Ferguson C, Parton RG, Tai AW. Rab18 binds to hepatitis C virus NS5A and promotes interaction between sites of viral replication and lipid droplets. PLoS Pathog. 2013;9(8): e1003513.
Ferguson D, Zhang J, Davis MA, Helsley RN, Vedin LL, Lee RG, et al. The lipid droplet-associated protein perilipin 3 facilitates hepatitis C virus-driven hepatic steatosis. J Lipid Res. 2017;58(2):420–32.
Hinson ER, Cresswell P. The antiviral protein, viperin, localizes to lipid droplets via its N-terminal amphipathic alpha-helix. Proc Natl Acad Sci U S A. 2009;106(48):20452–7.
Kloska A, Węsierska M, Malinowska M, Gabig-Cimińska M, Jakóbkiewicz-Banecka J. Lipophagy and lipolysis status in lipid storage and lipid metabolism diseases. Int J Mol Sci. 2020;21:17.
Pol A, Gross SP, Parton RG. Review: biogenesis of the multifunctional lipid droplet: lipids, proteins, and sites. J Cell Biol. 2014;204(5):635–46.
Yao J, Wei W, Wen J, Cao Y, Li H. The efficacy and mechanism of berberine in improving aging-related cognitive dysfunction: A study based on network pharmacology. Front Neurosci. 2023;17:1093180.
Zhu X, Bian H, Wang L, Sun X, Xu X, Yan H, et al. Berberine attenuates nonalcoholic hepatic steatosis through the AMPK-SREBP-1c-SCD1 pathway. Free Radic Biol Med. 2019;141:192–204.
Yu M, Alimujiang M, Hu L, Liu F, Bao Y, Yin J. Berberine alleviates lipid metabolism disorders via inhibition of mitochondrial complex I in gut and liver. Int J Biol Sci. 2021;17(7):1693–707.
Wang L, Xu BY, Sagada G, Ng WK, Chen K, Zhang JZ, et al. Dietary berberine regulates lipid metabolism in muscle and liver of black sea bream (Acanthopagrus schlegelii) fed normal or high-lipid diets. Br J Nutr. 2021;125(5):481–93.
Dai J, Huang X, Zhang C, Luo X, Cao S, Wang J, et al. Berberine regulates lipid metabolism via miR-192 in porcine oocytes matured in vitro. Vet Med Sci. 2021;7(3):950–9.
Liu YX, Fang XJ, Li Y, Bing L, Li Y, Fang J, et al. Berberine suppresses the migration and invasion of colon cancer cells by inhibition of lipogenesis through modulation of promyelocytic leukemia zinc finger-mediated sterol-regulatory element binding proteins cleavage-activating protein ubiquitination. J Pharm Pharmacol. 2022;74(9):1353–63.
Jyotshna KP, Mangiferin SK. A review of sources and interventions for biological activities. Biofactors. 2016;42(5):504–14.
Na L, Zhang Q, Jiang S, Du S, Zhang W, Li Y, et al. Mangiferin supplementation improves serum lipid profiles in overweight patients with hyperlipidemia: a double-blind randomized controlled trial. Sci Rep. 2015;5:10344.
Fomenko EV, Chi Y. Mangiferin modulation of metabolism and metabolic syndrome. BioFactors. 2016;42(5):492–503.
Sim MO, Lee HJ, Jeong DE, Jang JH, Jung HK, Cho HW. 6’-O-acetyl mangiferin from Iris rossii Baker inhibits lipid accumulation partly via AMPK activation in adipogenesis. Chem Biol Interact. 2019;311: 108755.
Niu Y, Li S, Na L, Feng R, Liu L, Li Y, et al. Mangiferin decreases plasma free fatty acids through promoting its catabolism in liver by activation of AMPK. PLoS ONE. 2012;7(1): e30782.
Tehrani SS, Goodarzi G, Panahi G, Zamani-Garmsiri F, Meshkani R. The combination of metformin with morin alleviates hepatic steatosis via modulating hepatic lipid metabolism, hepatic inflammation, brown adipose tissue thermogenesis, and white adipose tissue browning in high-fat diet-fed mice. Life Sci. 2023;323: 121706.
Zhang Q, Kong X, Yuan H, Guan H, Li Y, Niu Y. Mangiferin improved palmitate-induced-insulin resistance by promoting free fatty acid metabolism in HepG2 and C2C12 Cells via PPARα: mangiferin improved insulin resistance. J Diabetes Res. 2019;2019:2052675.
Guo F, Huang C, Liao X, Wang Y, He Y, Feng R, et al. Beneficial effects of mangiferin on hyperlipidemia in high-fat-fed hamsters. Mol Nutr Food Res. 2011;55(12):1809–18.
Ren K, Li H, Zhou HF, Liang Y, Tong M, Chen L, et al. Mangiferin promotes macrophage cholesterol efflux and protects against atherosclerosis by augmenting the expression of ABCA1 and ABCG1. Aging (Albany NY). 2019;11(23):10992–1009.
Rodriguez-Gonzalez JC, Hernández-Balmaseda I, Declerck K, Pérez-Novo C, Logie E, Theys C, et al. Antiproliferative, antiangiogenic, and antimetastatic therapy response by mangiferin in a syngeneic immunocompetent colorectal cancer mouse model involves changes in mitochondrial energy metabolism. Front Pharmacol. 2021;12: 670167.
Li J, Liu M, Yu H, Wang W, Han L, Chen Q, et al. Mangiferin improves hepatic lipid metabolism mainly through its metabolite-norathyriol by modulating SIRT-1/AMPK/SREBP-1c signaling. Front Pharmacol. 2018;9:201.
Yu S, Zhang W, Zhang L, Wu D, Sun P, Huang C, et al. MYB24 negatively regulates the biosynthesis of lignin and capsaicin by affecting the expression of key genes in the phenylpropanoid metabolism pathway in capsicum Chinense. Molecules. 2023;28:6.
Hochkogler CM, Lieder B, Schachner D, Heiss E, Schröter A, Hans J, et al. Capsaicin and nonivamide similarly modulate outcome measures of mitochondrial energy metabolism in HepG2 and 3T3-L1 cells. Food Funct. 2018;9(2):1123–32.
Baek J, Lee J, Kim K, Kim T, Kim D, Kim C, et al. Inhibitory effects of Capsicum annuum L water extracts on lipoprotein lipase activity in 3T3-L1 cells. Nutr Res Pract. 2013;7(2):96–102.
Hwang JT, Park IJ, Shin JI, Lee YK, Lee SK, Baik HW, et al. Genistein, EGCG, and capsaicin inhibit adipocyte differentiation process via activating AMP-activated protein kinase. Biochem Biophys Res Commun. 2005;338(2):694–9.
Lee MS, Kim CT, Kim IH, Kim Y. Effects of capsaicin on lipid catabolism in 3T3-L1 adipocytes. Phytother Res. 2011;25(6):935–9.
Chen J, Li L, Li Y, Liang X, Sun Q, Yu H, et al. Activation of TRPV1 channel by dietary capsaicin improves visceral fat remodeling through connexin43-mediated Ca2+ influx. Cardiovasc Diabetol. 2015;14:22.
Bort A, Sánchez BG, Mateos-Gómez PA, Díaz-Laviada I, Rodríguez-Henche N. Capsaicin targets lipogenesis in HepG2 cells through AMPK activation, AKT inhibition and PPARs regulation. Int J Mol Sci. 2019;20:7.
Li Q, Li L, Wang F, Chen J, Zhao Y, Wang P, et al. Dietary capsaicin prevents nonalcoholic fatty liver disease through transient receptor potential vanilloid 1-mediated peroxisome proliferator-activated receptor δ activation. Pflugers Arch. 2013;465(9):1303–16.
Jeong JY, Suresh S, Park MN, Jang M, Park S, Gobianand K, et al. Effects of capsaicin on adipogenic differentiation in bovine bone marrow mesenchymal stem cell. Asian-Australas J Anim Sci. 2014;27(12):1783–93.
Montanari T, Boschi F, Colitti M. Comparison of the effects of browning-inducing capsaicin on two murine adipocyte models. Front Physiol. 2019;10:1380.
Shin MK, Yang SM, Han IS. Capsaicin suppresses liver fat accumulation in high-fat diet-induced NAFLD mice. Anim Cells Syst (Seoul). 2020;24(4):214–9.
Pande S, Srinivasan K. Potentiation of hypolipidemic and weight-reducing influence of dietary tender cluster bean (Cyamopsis tetragonoloba) when combined with capsaicin in high-fat-fed rats. J Agric Food Chem. 2012;60(33):8155–62.
Mueller M, Beck V, Jungbauer A. PPARα activation by culinary herbs and spices. Planta Med. 2011;77(5):497–504.
Molloy JW, Calcagno CJ, Williams CD, Jones FJ, Torres DM, Harrison SA. Association of coffee and caffeine consumption with fatty liver disease, nonalcoholic steatohepatitis, and degree of hepatic fibrosis. Hepatology. 2012;55(2):429–36.
Su SH, Shyu HW, Yeh YT, Chen KM, Yeh H, Su SJ. Caffeine inhibits adipogenic differentiation of primary adipose-derived stem cells and bone marrow stromal cells. Toxicol In Vitro. 2013;27(6):1830–7.
Zheng X, Dai W, Chen X, Wang K, Zhang W, Liu L, et al. Caffeine reduces hepatic lipid accumulation through regulation of lipogenesis and ER stress in zebrafish larvae. J Biomed Sci. 2015;22:105.
Helal MG, Ayoub SE, Elkashefand WF, Ibrahim TM. Caffeine affects HFD-induced hepatic steatosis by multifactorial intervention. Hum Exp Toxicol. 2018;37(9):983–90.
Liu CW, Tsai HC, Huang CC, Tsai CY, Su YB, Lin MW, et al. Effects and mechanisms of caffeine to improve immunological and metabolic abnormalities in diet-induced obese rats. Am J Physiol Endocrinol Metab. 2018;314(5):E433–47.
Velickovic K, Wayne D, Leija HAL, Bloor I, Morris DE, Law J, et al. Caffeine exposure induces browning features in adipose tissue in vitro and in vivo. Sci Rep. 2019;9(1):9104.
Velickovic K, Lugo Leija HA, Bloor I, Law J, Sacks H, Symonds M, et al. Low temperature exposure induces browning of bone marrow stem cell derived adipocytes in vitro. Sci Rep. 2018;8(1):4974.
Lv Z, Xing K, Li G, Liu D, Guo Y. Dietary genistein alleviates lipid metabolism disorder and inflammatory response in laying hens with fatty liver syndrome. Front Physiol. 2018;9:1493.
Qin H, Song Z, Shaukat H, Zheng W. Genistein regulates lipid metabolism via estrogen receptor β and its downstream signal Akt/mTOR in HepG2 Cells. Nutrients. 2021;13:11.
Kim MH, Kang KS, Lee YS. The inhibitory effect of genistein on hepatic steatosis is linked to visceral adipocyte metabolism in mice with diet-induced non-alcoholic fatty liver disease. Br J Nutr. 2010;104(9):1333–42.
Seidemann L, Krüger A, Kegel-Hübner V, Seehofer D, Damm G. Influence of genistein on hepatic lipid metabolism in an in vitro model of hepatic steatosis. Molecules. 2021;26:4.
Xiao CWu, Wood CM, Weber D, Aziz SA, Mehta R, Griffin P, Cockell KA, et al. Dietary supplementation with soy isoflavones or replacement with soy proteins prevents hepatic lipid droplet accumulation and alters expression of genes involved in lipid metabolism in rats. Genes Nutr. 2014;9:1.
Tovar AR, Murguía F, Cruz CR, Hernández-Pando R, Fau - Aguilar-Salinas CA, Aguilar-Salinas Ca Fau - Pedraza-Chaverri J, Pedraza-Chaverri J Fau - Correa-Rotter R, et al. A soy protein diet alters hepatic lipid metabolism gene expression and reduces serum lipids and renal fibrogenic cytokines in rats with chronic nephrotic syndrome. J Nutr. 2002;132:9.
Kuiper GG, Lemmen JG, Carlsson B, Corton JC, Safe SH, van der Saag PT, et al. Interaction of estrogenic chemicals and phytoestrogens with estrogen receptor beta. Endocrinology. 1998;139(10):4252–63.
Nie Q, Xing M, Hu J, Hu X, Nie S, Xie M. Metabolism and health effects of phyto-estrogens. Crit Rev Food Sci Nutr. 2017;57(11):2432–54.
Tang G, Xu Y, Zhang C, Wang N, Li H, Feng Y. Green tea and epigallocatechin gallate (EGCG) for the management of nonalcoholic fatty liver diseases (NAFLD): insights into the role of oxidative stress and antioxidant mechanism. Antioxidants. 2021;10:7.
Yuan H, Li Y, Ling F, Guan Y, Zhang D, Zhu Q, et al. The phytochemical epigallocatechin gallate prolongs the lifespan by improving lipid metabolism, reducing inflammation and oxidative stress in high-fat diet-fed obese rats. Aging Cell. 2020;19(9): e13199.
Li Y, Wu S. Epigallocatechin gallate suppresses hepatic cholesterol synthesis by targeting SREBP-2 through SIRT1/FOXO1 signaling pathway. Mol Cell Biochem. 2018;448(1–2):175–85.
Li Y, Song Y, Zhao M, Guo Y, Yu C, Chen W, et al. A novel role for CRTC2 in hepatic cholesterol synthesis through SREBP-2. Hepatology (Baltimore, MD). 2017;66(2):481–97.
Lin L, Zeng L, Liu A, Yuan D, Peng Y, Zhang S, et al. Role of epigallocatechin gallate in glucose, lipid, and protein metabolism and L-theanine in the metabolism-regulatory effects of epigallocatechin gallate. Nutrients. 2021;13:11.
Ding H, Li Y, Li W, Tao H, Liu L, Zhang C, et al. Epigallocatechin-3-gallate activates the AMP-activated protein kinase signaling pathway to reduce lipid accumulation in canine hepatocytes. J Cell Physiol. 2021;236(1):405–16.
Kim HS, Montana V, Jang HJ, Parpura V, Kim JA. Epigallocatechin gallate (EGCG) stimulates autophagy in vascular endothelial cells: a potential role for reducing lipid accumulation. J Biol Chem. 2013;288(31):22693–705.
Li F, Gao C, Yan P, Zhang M, Wang Y, Hu Y, et al. EGCG reduces obesity and white adipose tissue gain partly through AMPK activation in mice. Front Pharmacol. 2018;9:1366.
Huang JB, Zhang Y, Zhou YB, Wan XC, Zhang JS. Effects of epigallocatechin gallate on lipid metabolism and its underlying molecular mechanism in broiler chickens. J Anim Physiol Anim Nutr. 2015;99(4):719–27.
Naveed M, Hejazi V, Abbas M, Kamboh AA, Khan GJ, Shumzaid M, et al. Chlorogenic acid (CGA): a pharmacological review and call for further research. Biomed Pharmacother. 2018;97:67–74.
Zhang LT, Chang CQ, Liu Y, Chen ZM. Effect of chlorogenic acid on disordered glucose and lipid metabolism in db/db mice and its mechanism. Zhongguo Yi Xue Ke Xue Yuan Xue Bao. 2011;33(3):281–6.
Gao X, Zhu Z, Bao Y, Li Y, Zhu W, He X, et al. Chrysanthemum morifolium Ramat extract and probiotics combination ameliorates metabolic disorders through regulating gut microbiota and PPARα subcellular localization. Chinese Med. 2024;19:1.
Sudeep HV, Venkatakrishna K, Patel D, Shyamprasad K. Biomechanism of chlorogenic acid complex mediated plasma free fatty acid metabolism in rat liver. BMC Complement Altern Med. 2016;16:274.
Cho AS, Jeon SM, Kim MJ, Yeo J, Seo KI, Choi MS, et al. Chlorogenic acid exhibits anti-obesity property and improves lipid metabolism in high-fat diet-induced-obese mice. Food Chem Toxicol. 2010;48(3):937–43.
Zhou Y, Ruan Z, Wen Y, Yang Y, Mi S, Zhou L, et al. Chlorogenic acid from honeysuckle improves hepatic lipid dysregulation and modulates hepatic fatty acid composition in rats with chronic endotoxin infusion. J Clin Biochem Nutr. 2016;58(2):146–55.
Mubarak A, Hodgson JM, Considine MJ, Croft KD, Matthews VB. Supplementation of a high-fat diet with chlorogenic acid is associated with insulin resistance and hepatic lipid accumulation in mice. J Agric Food Chem. 2013;61(18):4371–8.
Yan Y, Li Q, Shen L, Guo K, Zhou X. Chlorogenic acid improves glucose tolerance, lipid metabolism, inflammation and microbiota composition in diabetic db/db mice. Front Endocrinol (Lausanne). 2022;13:1042044.
Wang Y, Peng S, Mei Z, Jin C, Kang J, Xiang M, et al. Chlorogenic acid inhibits forming of diabetes mellitus in rats induced by high-fat high-sucrose and streptozotocin. Pak J Pharm Sci. 2020;33(3):1063–72.
Peng SG, Pang YL, Zhu Q, Kang JH, Liu MX, Wang Z. Chlorogenic acid functions as a novel agonist of PPARγ2 during the differentiation of mouse 3T3-L1 Preadipocytes. Biomed Res Int. 2018;2018:8594767.
Yao R, Wang M, Zhao Y, Ji Q, Feng X, Bai L, et al. Chlorogenic acid enhances PPARγ -mediated lipogenesis through preventing Lipin 1 nuclear translocation in Staphylococcus aureus -exposed bovine mammary epithelial cells. Biochimica Et Biophysica Acta-Mol Cell Biol Lipids. 2023;1868:11.
Choi YR, Kim YS, Kim MJ. Cinnamyl alcohol attenuates adipogenesis in 3T3-L1 cells by arresting the cell cycle. Int J Mol Sci. 2024;25:2.
Arumugam MK, Paal MC, Donohue TM Jr, Ganesan M, Osna NA, Kharbanda KK. Beneficial effects of betaine a comprehensive review. Biology. 2021;10:6.
Yang W, Huang L, Gao J, Wen S, Tai Y, Chen M, et al. Betaine attenuates chronic alcohol-induced fatty liver by broadly regulating hepatic lipid metabolism. Mol Med Rep. 2017;16(4):5225–34.
Dou X, Xia Y, Chen J, Qian Y, Li S, Zhang X, et al. Rectification of impaired adipose tissue methylation status and lipolytic response contributes to hepatoprotective effect of betaine in a mouse model of alcoholic liver disease. Br J Pharmacol. 2014;171(17):4073–86.
Yang W, Gao J, Tai Y, Chen M, Huang L, Wen S, et al. Betaine attenuates alcohol-induced pancreatic steatosis. Pancreas. 2016;45(6):836–45.
Mackowiak B, Fu Y, Maccioni L, Gao B. Alcohol-associated liver disease. J Clin Investigat. 2024;134:3.
Liu J, Song R, Su S, Qi N, Li Q, Xie Z, et al. Betaine promotes fat accumulation and reduces injury in landes goose hepatocytes by regulating multiple lipid metabolism pathways. Animals. 2022;12:12.
Xu L, Huang D, Hu Q, Wu J, Wang Y, Feng J. Betaine alleviates hepatic lipid accumulation via enhancing hepatic lipid export and fatty acid oxidation in rats fed with a high-fat diet. Br J Nutr. 2015;113(12):1835–43.
Adjoumani JY, Wang K, Zhou M, Liu W, Zhang D. Effect of dietary betaine on growth performance, antioxidant capacity and lipid metabolism in blunt snout bream fed a high-fat diet. Fish Physiol Biochem. 2017;43(6):1733–45.
Chen W, Zhang X, Xu M, Jiang L, Zhou M, Liu W, et al. Betaine prevented high-fat diet-induced NAFLD by regulating the FGF10/AMPK signaling pathway in ApoE(-/-) mice. Eur J Nutr. 2021;60(3):1655–68.
Zhou P, Xie W, He S, Sun Y, Meng X, Sun G, et al. Ginsenoside Rb1 as an anti-diabetic agent and its underlying mechanism analysis. Cells. 2019;8:3.
Fan Q, Xi P, Tian D, Jia L, Cao Y, Zhan K, et al. Ginsenoside Rb1 facilitates browning by repressing Wnt/β-catenin signaling in 3T3-L1 adipocytes. Med Sci Monit. 2021;27: e928619.
Hou Y, Gu D, Peng J, Jiang K, Li Z, Shi J, et al. Ginsenoside Rg1 regulates liver lipid factor metabolism in NAFLD model rats. ACS Omega. 2020;5(19):10878–90.
Qiao L, Zhang X, Liu M, Liu X, Dong M, Cheng J, et al. Ginsenoside Rb1 enhances atherosclerotic plaque stability by improving autophagy and lipid metabolism in macrophage foam cells. Front Pharmacol. 2017;8:727.
Hwang JT, Lee MS, Kim HJ, Sung MJ, Kim HY, Kim MS, et al. Antiobesity effect of ginsenoside Rg3 involves the AMPK and PPAR-gamma signal pathways. Phytotherapy Res. 2009;23(2):262–6.
Quan HY, Yuan HD, Jung MS, Ko SK, Park YG, Chung SH. Ginsenoside Re lowers blood glucose and lipid levels via activation of AMP-activated protein kinase in HepG2 cells and high-fat diet fed mice. Int J Mol Med. 2012;29(1):73–80.
Li G, Xu Y, Gao Q, Guo S, Zu Y, Wang X, et al. Ginsenosides restore lipid and redox homeostasis in mice with intrahepatic cholestasis through SIRT1/AMPK pathways. Nutrients. 2022;14:19.
Oh JM, Chun S. Ginsenoside CK inhibits the early stage of adipogenesis via the AMPK, MAPK, and AKT signaling pathways. Antioxidants. 2022;11:10.
Huang YC, Lin CY, Huang SF, Lin HC, Chang WL, Chang TC. Effect and mechanism of ginsenosides CK and Rg1 on stimulation of glucose uptake in 3T3-L1 adipocytes. J Agric Food Chem. 2010;58(10):6039–47.
Hwang JT, Lee MS, Kim HJ, Sung MJ, Kim HY, et al. Antiobesity effect of ginsenoside Rg3 involves the AMPK and PPAR-gamma signal pathways. Phytother Res. 2009;23:2.
Lee S, Lee MS, Kim CT, Kim IH, Kim Y. Ginsenoside Rg3 reduces lipid accumulation with AMP-activated protein kinase (AMPK) activation in HepG2 cells. Int J Mol Sci. 2012;13(5):5729–39.
Gu W, Kim KA, Kim DH. Ginsenoside Rh1 ameliorates high fat diet-induced obesity in mice by inhibiting adipocyte differentiation. Biol Pharm Bull. 2013;36(1):102–7.
Liu H, Liu M, Jin Z, Yaqoob S, Zheng M, Cai D, et al. Ginsenoside Rg2 inhibits adipogenesis in 3T3-L1 preadipocytes and suppresses obesity in high-fat-diet-induced obese mice through the AMPK pathway. Food Funct. 2019;10(6):3603–14.
Gao Y, Zhang S, Li J, Zhao J, Xiao Q, Zhu Y, et al. Effect and mechanism of ginsenoside Rg1-regulating hepatic steatosis in HepG2 cells induced by free fatty acid. Biosci Biotechnol Biochem. 2020;84(11):2228–40.
Siraj FM, SathishKumar N, Kim YJ, Kim SY, Yang DC. Ginsenoside F2 possesses anti-obesity activity via binding with PPARγ and inhibiting adipocyte differentiation in the 3T3-L1 cell line. J Enzyme Inhib Med Chem. 2015;30(1):9–14.
Liu Z, Liao W, Yin X, Zheng X, Li Q, Zhang H, et al. Resveratrol-induced brown fat-like phenotype in 3T3-L1 adipocytes partly via mTOR pathway. Food Nutr Res. 2020;64:1.
Wang S, Liang X, Yang Q, Fu X, Zhu M, Rodgers BD, et al. Resveratrol enhances brown adipocyte formation and function by activating AMP-activated protein kinase (AMPK) α1 in mice fed high-fat diet. Mol Nutr Food Res. 2017;61:4.
Cho SJ, Jung UJ, Choi MS. Differential effects of low-dose resveratrol on adiposity and hepatic steatosis in diet-induced obese mice. Br J Nutr. 2012;108(12):2166–75.
Jing Y, Hu T, Lin C, Xiong Q, Liu F, Yuan J, et al. Resveratrol downregulates PCSK9 expression and attenuates steatosis through estrogen receptor α-mediated pathway in L02 cells. Eur J Pharmacol. 2019;855:216–26.
Zhou R, Yi L, Ye X, Zeng X, Liu K, Qin Y, et al. Resveratrol ameliorates lipid droplet accumulation in liver through a SIRT1/ ATF6-dependent mechanism. Cell Physiol Biochem. 2018;51(5):2397–420.
Tang LY, Chen Y, Rui BB, Hu CM. Resveratrol ameliorates lipid accumulation in HepG2 cells, associated with down-regulation of lipin1 expression. Can J Physiol Pharmacol. 2016;94(2):185–9.
Wang C, Yuan Y, Li L, Zhang XM, Li J, Hu PZ, et al. Effects of resveratrol on the morphology of lipid droplets and the expression of lipid droplet-associated proteins in mouse primary hepatocytes. Xi Bao Yu Fen Zi Mian Yi Xue Za Zhi. 2012;28(9):911–4.
Nishikawa K, Iwaya K, Kinoshita M, Fujiwara Y, Akao M, Sonoda M, et al. Resveratrol increases CD68+ Kupffer cells colocalized with adipose differentiation-related protein and ameliorates high-fat-diet-induced fatty liver in mice. Mol Nutr Food Res. 2015;59(6):1155–70.
Ma Q, Shen M, Wu J, Ye C, Tan Y. Mechanism research of DHEA treatment improving diminished ovarian reserve by attenuating the AMPK-SIRT1 signaling and mitophagy. Reproductive Sci. 2024;1:14.
Zhou R, Yi L, Ye X, Zeng X, Liu K, Qin Y, et al. Resveratrol ameliorates lipid droplet accumulation in liver through a SIRT1/ATF6-dependent mechanism. Cell Physiol Biochem. 2018;51(5):2397–420.
Chang N, Li J, Lin S, Zhang J, Zeng W, Ma G, et al. Emerging roles of SIRT1 activator, SRT2104, in disease treatment. Sci Rep. 2024;14(1):5521.
Mehdi F, Keihan GS, Asadollah AS, Effat F. The effects of resveratrol, metformin, cold and strength training on the level of perilipin 5 in the heart, skeletal muscle and brown adipose tissues in mouse. Cell Biochem Biophys. 2018;76(4):471–6.
van Polanen N, Zacharewicz E, de Ligt M, Timmers S, Moonen-Kornips E, Schaart G, et al. Resveratrol-induced remodelling of myocellular lipid stores: a study in metabolically compromised humans. Physiol Rep. 2021;9(2): e14692.
Calle RA, Amin NB, Carvajal-Gonzalez S, Ross TT, Bergman A, Aggarwal S, et al. ACC inhibitor alone or co-administered with a DGAT2 inhibitor in patients with non-alcoholic fatty liver disease: two parallel, placebo-controlled, randomized phase 2a trials. Nat Med. 2021;27(10):1836–48.
Yue S, Li J, Lee SY, Lee HJ, Shao T, Song B, et al. Cholesteryl ester accumulation induced by PTEN loss and PI3K/AKT activation underlies human prostate cancer aggressiveness. Cell Metab. 2014;19(3):393–406.
Donnerer J, Amann R, Schuligoi R, Lembeck F. Absorption and metabolism of capsaicinoids following intragastric administration in rats. Naunyn Schmiedebergs Arch Pharmacol. 1990;342(3):357–61.
Kawada T, Watanabe T, Katsura K, Takami H, Iwai K. Formation and metabolism of pungent principle of capsicum fruits. XV. Microdetermination of capsaicin by high-performance liquid chromatography with electrochemical detection. J Chromato A. 1985;329:99–105.
Yu Q, Li M, Chen H, Xu L, Cheng J, Lin G, et al. The discovery of berberine erythrocyte-hemoglobin self-assembly delivery system: a neglected carrier underlying its pharmacokinetics. Drug Delivery. 2022;29(1):856–70.
Coppinger C, Pomales B, Movahed MR, Marefat M, Hashemzadeh M. Berberine: a multi-target natural PCSK9 inhibitor with the potential to treat diabetes, alzheimer’s, cancer and cardiovascular disease. Curr Rev Clin Exp Pharmacol. 2024;19:4.
Hao S, Xiao Y, Lin Y, Mo Z, Chen Y, Peng X, et al. Chlorogenic acid-enriched extract from Eucommia ulmoides leaves inhibits hepatic lipid accumulation through regulation of cholesterol metabolism in HepG2 cells. Pharm Biol. 2016;54(2):251–9.
Hong Y, Feng J, Dou Z, Sun X, Hu Y, Chen Z, et al. Berberine as a novel ACSL4 inhibitor to suppress endothelial ferroptosis and atherosclerosis. Biomed Pharmacother. 2024;177:117081.
Hu YH, Han J, Wang L, Shi C, Li Y, Olatunji OJ, et al. α-Mangostin Alleviated Inflammation in Rats With Adjuvant-Induced Arthritis by Disrupting Adipocytes-Mediated Metabolism-Immune Feedback. Front Pharmacol. 2021;12:692806.
Sanchez MB, Miranda-Perez E, Verjan JCG, de Los Angeles Fortis Barrera M, Perez-Ramos J, Alarcon-Aguilar FJ. Potential of the chlorogenic acid as multitarget agent: Insulin-secretagogue and PPAR α/γ dual agonist. Biomed Pharmacother. 2017;94:169–75.
Yao R, Wang M, Zhao Y, Ji Q, Feng X, Bai L, et al. Chlorogenic acid enhances PPARγ-mediated lipogenesis through preventing Lipin 1 nuclear translocation in Staphylococcus aureus-exposed bovine mammary epithelial cells. Biochim Biophys Acta Mol Cell Biol Lipids. 2023;1868(11):159396.
Yuanyuan Z, Huaizhen L. Mechanistic research into the effects of the Jianpi Xiaozhi formula on liver injury in diabetic rats. Evid Based Complement Alternat Med. 2022;2022:7490747.
Zhang L, Zheng YL, Hu RH, Zhu L, Hu CC, Cheng F, et al. Annexin A1 mimetic peptide AC2-26 inhibits sepsis-induced cardiomyocyte apoptosis through LXA4/PI3K/AKT signaling pathway. Curr Med Sci. 2018;38(6):997–1004.
Zhong H, Chen K, Feng M, Shao W, Wu J, Chen K, et al. Genipin alleviates high-fat diet-induced hyperlipidemia and hepatic lipid accumulation in mice via miR-142a-5p/SREBP-1c axis. FEBS J. 2018;285(3):501–17.
Acknowledgements
Not applicable.
Funding
This study was supported by the National Natural Science Foundation of China (No. 82174159) and the Fifth Batch of National Traditional Chinese Medicine Excellent Clinical Talents Training Project. (Announcement from the Personnel and Education Department of the National Administration of Traditional Chinese Medicine. No. 2022–1).
Author information
Authors and Affiliations
Contributions
Xinyue Jiang: Writing-Original Draft, Conceptualization. Methodology. Hongzhan Wang: Visualization, Conceptualization. Methodology. Kexin Nie: Methodology. Yang Gao: Visualization. Shen Chen: Visualization. Yueheng Tang: Data Curation. Zhi Wang: Resources. Hao Su: Supervision. Hui Dong: Writing-Review & Editing, Supervision, Funding acquisition.
Corresponding author
Ethics declarations
Ethics approval and consent to participate
Not applicable.
Consent for publication
Not applicable.
Competing interests
The authors declare no competing interests.
Additional information
Publisher's Note
Springer Nature remains neutral with regard to jurisdictional claims in published maps and institutional affiliations.
Rights and permissions
Open Access This article is licensed under a Creative Commons Attribution 4.0 International License, which permits use, sharing, adaptation, distribution and reproduction in any medium or format, as long as you give appropriate credit to the original author(s) and the source, provide a link to the Creative Commons licence, and indicate if changes were made. The images or other third party material in this article are included in the article's Creative Commons licence, unless indicated otherwise in a credit line to the material. If material is not included in the article's Creative Commons licence and your intended use is not permitted by statutory regulation or exceeds the permitted use, you will need to obtain permission directly from the copyright holder. To view a copy of this licence, visit http://creativecommons.org/licenses/by/4.0/. The Creative Commons Public Domain Dedication waiver (http://creativecommons.org/publicdomain/zero/1.0/) applies to the data made available in this article, unless otherwise stated in a credit line to the data.
About this article
Cite this article
Jiang, X., Wang, H., Nie, K. et al. Targeting lipid droplets and lipid droplet-associated proteins: a new perspective on natural compounds against metabolic diseases. Chin Med 19, 120 (2024). https://doiorg.publicaciones.saludcastillayleon.es/10.1186/s13020-024-00988-w
Received:
Accepted:
Published:
DOI: https://doiorg.publicaciones.saludcastillayleon.es/10.1186/s13020-024-00988-w